
This is a test
- English
- ePUB (mobile friendly)
- Available on iOS & Android
eBook - ePub
Book details
Book preview
Table of contents
Citations
About This Book
There is no doubt: climate change is happening, and mankind is increasingly to blame. Climate Change: The Point of No Return provides a solid basis for the current discussion about climate change, by addressing the arguments from both sides of the debate and offering an objective evaluation of the facts. Using the latest scientific information about the causes of the global climate change, Professor Latif presents the likely scenario that will face us if we don't dedicate ourselves to a course of sustainable development, and offers concrete options for action.
Frequently asked questions
At the moment all of our mobile-responsive ePub books are available to download via the app. Most of our PDFs are also available to download and we're working on making the final remaining ones downloadable now. Learn more here.
Both plans give you full access to the library and all of Perlegoâs features. The only differences are the price and subscription period: With the annual plan youâll save around 30% compared to 12 months on the monthly plan.
We are an online textbook subscription service, where you can get access to an entire online library for less than the price of a single book per month. With over 1 million books across 1000+ topics, weâve got you covered! Learn more here.
Look out for the read-aloud symbol on your next book to see if you can listen to it. The read-aloud tool reads text aloud for you, highlighting the text as it is being read. You can pause it, speed it up and slow it down. Learn more here.
Yes, you can access Climate Change by Mojib Latif in PDF and/or ePUB format, as well as other popular books in Biological Sciences & Science General. We have over one million books available in our catalogue for you to explore.
Information
The Scientific Principles
1 The Climate and the Earth System
1.1 Weather and Climate
The difference between weather and climate can be explained in one sentence, uttered by Larry Gates, one of the American pioneers of climate research, at an event held by the World Meteorological Organization (WMO) in the early 1980s: âClimate is what you expect, weather is what you get.â So there is a fundamental difference between weather and climate. Weather research is concerned with the generation, propagation and prediction of individual weather elements, such as a certain mid-latitudinal storm or a hurricane, while climate research is interested in the entirety of mid-latitudinal storms and hurricanes, and addresses such questions as how many storms or hurricanes there will be next year, or whether they will become more frequent or intensify as a consequence of global warming. With the term âweatherâ we thus designate the short-term occurrences in the atmosphere, while the term âclimateâ refers to longer periods of time. The World Meteorological Organization defines climate as the statistics of weather over a period that is long enough to allow these statistical properties to be determined. While weather describes the physical state of the atmosphere at a certain point of time at a certain location, a description of climate is not complete until it includes the probability for deviations from the mean, and thus the probability of extreme weather events occurring, such as the flooding of the Elbe river in 2002 or the drought in Germany the following year. Generally a span of thirty years is used as the reference period for a description of the climate.
The term âclimateâ is derived from klinein, the Greek word for âtilt,â since summer and winter are consequences of the inclination of the Earthâs axis relative to the plane containing the Earthâs orbit around the sun, known as the ecliptic. Currently this tilt measures 23.5°, meaning that the Northern Hemisphere is irradiated more strongly during the northern summer and the Southern Hemisphere more strongly during the northern winter. For the most part, the usual geographical classification into climatic zones follows the resulting seasonal cycle of meteorological values such as temperature and precipitation. The tilt of the Earthâs axis, along with other so-called orbital parameters, vary over the course of millennia and are in part responsible for the fact that the history of the Earth has seen strong climatic excursions (see Section 3.5).
The different seasonal and annual mean levels of the sunâs irradiation at the equator and the poles results in great regional differences in the surface temperature of the Earth. The resulting horizontal temperature differences in the lower atmosphere lead to differences in atmospheric pressure. Finally, the resulting force of pressure, together with the influence of gravity and the rotation of the Earth, causes the winds. The average three-dimensional wind pattern is called the âgeneral circulationâ of the atmosphere. The atmosphere is not an isolated system, however, but interacts with other components of the earth system: with the hydrosphere (oceans and the water cycle on continents and in the atmosphere), the cryosphere (ice and snow), the biosphere (animals and plants), the pedosphere (soil) and the lithosphere (rock). These components define the climate system (see Figure 1), and they all move at completely different speeds and exhibit drastically different heat conductivities and heat capacities.

The dynamics of the climate system and the climate statistics that follow from it are thus characterized by the extremely different time scales of its individual components (see Figure 2). While the lower atmosphere adapts to conditions at the surface in just hours, the deep ocean circulation takes many centuries to adjust to changed boundary conditions like a modified composition of the atmosphere, and a large ice sheet like the Antarctic ice sheet requires many millennia for the process. Changes in the climate can emerge from interactions within a single climate component, or from interactions among the individual components, for instance between the ocean and the atmosphere. Changes can also be triggered externally by such events as a change in solar irradiation, by volcanic eruptions, or by a change in the composition of the Earthâs atmosphere. In the past hundred years man has taken on ever greater importance for the climate, by emitting into the atmosphere trace gases affecting the climate, which changes the atmospheric radiation budget, and thus contributes to global warming on the Earth.

1.2 The Oceans
Figure 2 shows a diagram of the components active in the climate system. It illustrates the corresponding time scales, ranging from hours up to millions of years. The âfastestâ component is the atmosphere, in which the significant energetic processes (such as the sequence of high and low pressure systems in our latitudes) exhibit a typical time scale of several days. The water cycle, which makes a crucial contribution to atmospheric energetics by means of the heat flows arising from evaporation and precipitation, is estimated to have a period of about 10 days. On the opposite end of the scale in Figure 2 are the ice sheets of Greenland and the Antarctic, whose characteristic time scales are in the range of many millennia. Fluctuations of this kind correspond to the rhythms of the global warm and cold periods (e.g. ice ages), which occur at intervals of between 10,000 and many 100,000 years.
Between the two extremes in Figure 2 lies the ocean, divided into the layers close to the surface and the deep sea. The former react over periods of weeks and months, as the currents and stratification fluctuate due to direct links between the surface and the varying atmospheric fields of wind, temperature, radiation and precipitation. Changes in the deep sea, by contrast, depend on fluctuations in the surface conditions in limited regions of the polar and subpolar latitudes. Because of the huge amounts of water involved, they play out over periods of time ranging from decades to several centuries. Since global warming refers to precisely this range of the time scales, the oceans play a very important role in anthropogenically induced climate change.
In continuous media, different time scales are also associated with different spatial scales. The spatial scale significant for atmospheric energetics occurs at around 1000 km. This corresponds to the typical extension of the high and low pressure systems in the atmosphere. In the ocean the most energetic current and stratification changes extend to distances of around 10 to 100 km. These changes take the form of âmesoscaleâ vortexes (eddies), the oceanic analog to atmospheric highs and lows. The âfastâ atmospheric processes (which take several days) have a comparatively larger extension (1000 km) than the âslowâ processes (which can last for months) within the mixed layer of the ocean (the âmixed layerâ is the top layer, well mixed by waves, tides and weather events). This makes the observation of the most energetic oceanic movements much more difficult than that of the atmospheric ones. Observations show that mesoscale eddies exist even at great depths of several thousand meters. Because these, too, play an important role for long-term climate fluctuations, they must be subjected to constant observation and simulated in models.
The central role of water in the climate system is based on the asymmetrical structure of the water molecule, which makes it an electric dipole, encouraging the temperature-dependent aggregation of water molecules. This is why water has the anomalous property of possessing its greatest density at 4°C, such that its solid phase, ice, floats. We find huge amounts of floating ice, known as âsea ice,â in the Arctic and Antarctic. Because its molecules cohere, water reacts inertly to both warming and cooling, lending it the highest heat capacity of all liquid and solid substances (with the exception of ammonia), and shifting its boiling and freezing points to 100°C and 0°, respectively, from the â80°C and -110°C that would be expected if the water molecule had a symmetrical structure.
Moreover, water is an extremely effective solvent. The relative weight component of the dissolved substances is called âsalinity,â and averages 3.47% in the worldâs oceans (in oceanography salinity is usually given in tenths of a percent). This salinity significantly changes the above mentioned properties of water. For instance, at a salinity of 3.47% the temperature of the maximum density shifts to â3.8°C, and drops below the freezing point of â1.9°C. This means that the phenomenon of âconvectionâ can take place while the ocean cools until ice forms: cooled water sinks, warmer water rises from the depths, releasing its heat content to the atmosphere, and then sinks to the depths after absorbing atmospheric gases. Convection plays a major role in cloud formation in the atmosphere as well. However, it also takes place in the interior of the Earth and is thus one of the most important processes propelling movement throughout the entire Earth system.
With regard to circulation and stratification (the vertical structure of density), primary stimulation of the ocean can take place only from the surface. In the case of a wind forcing, the interplay of friction, the deflective force of the Earthâs rotation (Coriolis force) and the shape of the ocean basin lead to the system of surface currents known from any atlas, whose intensity decreases with depth. Familiar examples include the Gulf Stream in the Atlantic and the Kuroshio in the Pacific. Since the wind fields are neither temporally nor spatially homogenous, the mass transport associated with the currents causes regional mass surpluses or deficits. The rise and drop in the ocean surface associated with these effects drive not only long period waves and horizontal currents in the interior of the ocean, but also vertical motions. Upwelling regions are frequently nutrient-rich areas and thus tend to feature strong biological production.
As the ocean surface warms or cools, or as salinity changes due to evaporation, precipitation or the formation of sea ice, water masses of different density are created. In the Tropics and Subtropics, the surface layers are warmed constantly in the annual mean. The correspondingly light surface water floats as a warm top layer â with a temperature above 10°C â on the water masses located below. The strong temperature difference (density difference) quite effectively prevents the warming from penetrating the deeper layers, so that the top layer remains relatively thin, with a thickness between 100 and 800 meters. In the subpolar and polar areas the average heat deficit results in an increase in density, reinforced by the salt released when seawater freezes in certain regions; on average, the effect is deep convection.
Correspondingly, the oceans of higher latitudes are characterized by low stratification; signals from the surface can reach the interior of the ocean quite effectively and vice versa. The cold water from the polar oceans â with a temperature of less than 10°C â penetrates under the warm top layer into the interior: This launches a circulation driven by temperature and salt (the thermohaline circulation), for the cold water flowing toward the equator as it sinks to the depths is compensated for by the poleward transport of warm water in the top layer. The resulting oceanic heat transport on the Northern Hemisphere measures a maximum of 3.0 * 1015 Watts; this amount, combined with the heat transport of the atmosphere, is needed to balance the Northern Hemisphereâs radiation budget of 5.5 * 1015 Watts. Applied to the Atlantic, this thermohaline circulation is generally referred in media to as the âGulf Stream circulationâ or simply the âGulf Stream.â
The thermohaline circulation is propelled by convection processes in the higher latitudes. The surface conditions define the characteristics of the cold water masses created. These characteristics can be followed along their path toward the deep sea by measuring their temperatures, salinities, and densities or the content of oxygen and other trace gases. Most of the deepest water masses are of Antarctic origin (known as Antarctic Bottom Water). The water masses of Arctic origin are slightly less dense and thus stratified over the bottom layer. Since only the Atlantic Ocean extends to the higher latitudes on the Northern Hemisphere, this water begins its circulation in the Greenland Sea and the Labrador Sea (North Atlantic Deep Water). Figure 3 shows a diagram of its global spreading and the compensatory return current in the top layer. This circulation, also known as the âglobal conveyor beltâ or the âglobal overturning circulation,â has a cycle lasting several hundred up to a thousand years, as estimated using dating methods measuring the number of radioactive carbon isotopes in deep water (isotopes are different types of atoms of the same chemical element each having different atomic mass).
The longest part of this cycle takes place in the cold path of the conveyor belt in the deep layers, where typical velocities amount to just 1 to 3 km per day. The great masses of water involved in this circulation, amounting to around 0.4 billion kmÂł (around one third of the entire amount of seawater) transported at a rate of around 20 million mÂł/s, combined with this waterâs heat storage capacity and high solubility, makes the thermohaline circulation one of the most important long-term warehouses in the climate system. The substances stored in this warehouse include some of the greenhouse gas carbon dioxide emitted by man. Because the biogeochemical processes involved are still largely unknown, it is not possible to determine the capacity of the oceanic warehouse accurately. Therefore it is uncertain how long the ocean will continue to curb the atmospheric rise of anthropogenic CO2 and thus moderate the global warming caused by an increased greenhouse effect (see section 2.4).
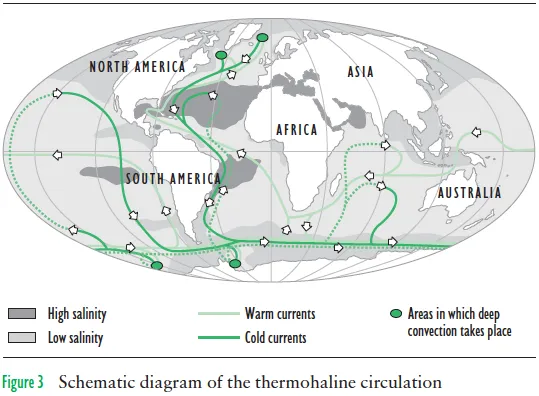
A crucial question pertaining to regional and global climate change concerns the stability of the thermohaline circulation. This issue trains on the changeability of convection in the higher latitudes. On the one hand, convection could be reduced by warming as a consequence of the anthropogenic greenhouse effect. On the other hand, in low-temperature areas salinity variations are very important, like those caused by changes in continental ice sheets, in precipitation and in river runoff from the continents into the North Atlantic â processes that also can be influenced by anthropogenic climate change. This complex of issues, known in the media as the âGulf Stream problem,â is a matter of intensive discussion not only in science, but in the general public as well. Even Hollywood took on this issue in the movie The Day After Tomorrow (see section 4.5). Suffice it to say at this juncture that a collapse of the thermohaline circulation will not trigger a new ice age.
1.3 Sea Ice
Sea ice is part of the cryosphere. This sphere encompasses all forms of snow and ice, making it the second largest component of the climate system in terms of mass and heat capacity. The significant components of the cryosphere are snow, sea ice, mountain glaciers, the ice shelves and the continental ice sheets. Today ice permanently covers 10% of the landâs surface (14.8 million km2) and around 6.5% of the oceans in the annual mean (22.5 million km2). With an area of 1.5 million km2, the ice shelves, most of which are in Antarctica, cover a significantly smaller ocean surface than does sea ice. However, the volume of the ice shelf is around 0.66 million km3, over ten times greater than that of sea ice. All mountain glaciers and smaller ice caps together have a volume of around 0.18 million km3.
Variations in the extent, solidity, thickness and drift of sea ice arise through dynamic and thermodynamic processes. The thermodynamics of sea ice, i.e. freezing and melting, is influenced by radiation processes and by the turbulent fluxes of latent and sensible heat. Sea ice drift is caused by wind stress, ocean current, Coriolis force and internal stresses in sea ice (deformation). The internal stresses are determined using rheological models. Rheology is a discipline of solid state physics and continuum mechanics that deals with the flow and deformation of materials.
Changes in the sea ice boundary are among the most important properties of climate fluctuations in the Polar Regions. On the geophysical scale, sea ice is a thin, broken layer on the polar oceans, which is moved by wind and ocean currents and whose thickness and extent is affected by thermodynamic processes. Sea ice constitutes the boundary between the two much larger components of the Earth system, atmosphere and ocean, and thus exerts considerable influence on their interaction. In March sea ice covers 5% of the ocean surface; in September 8%. In the Arctic Ocean the average thickness is 3 m, in the Antarctic, 1 m. Since sea ice, even if it is not covered by snow, has quite a high albedo (capacity to reflect solar radiation) and thus a significant influence on the radiation budget, it plays an important role in the climate system. This role is further increased by the fact that its insulating effect prevents any direct, turbulent exchange of momentum and heat between the ocean and the atmosphere. Thus the atmosphere is considerably colder above the surface of sea ice than over the open sea. The occurrence of sea ice not only cools the air in the Polar Regions, but also increases the meridional temperature gradient at the Earthâs surface â the temperature contrast between the Tropics and the Polar Regions â and thus intensifies the westerly winds in the mid-latitudes.
Sea ice influences the ocean as well as the atmosphere. The insulation of the ocean from heat losses to the cold atmosphere, and the change in stress at the ocean surface through losses in momentum due to the deformation of sea ice, are two important processes conditioned both by the intensive reduction in turbulent exchange and by the behavior of sea ice as a viscous-plastic solid flowing in two dimensions, driven by wind and ocean currents. Another important effect is the influence of sea ice on convection in the ocean and thus on the formation of deep and bottom water. Sea ice therefore also plays an important role in large-scale ocean circulation, especially for the thermohaline circulation. The average salinity of sea water is 3.47%, but that of sea ice is only around 0.5â°. When sea water freezes, a considerable quantity of salt is released into the ocean. This raises the density of sea water, making it heavier, and can trigger convection. The process of sea ice formation supports the generation of dense sea water in the Polar Regions, which can sink to deeper ocean layers thereby affecting the ocean circulation.
Some climate fluctuations on the time scale of decades are explained in the literature by means of the concept of âice-ocean oscillators.â For instance, imagine a fluctuation that includes the thermohaline circulation, in which increased ice formation leads to higher salinity and thus density of the water in the North Atlantic. The result is more convection at higher latitudes, causing the thermohaline circulation to intensify. Thus more heat is transported northward in the North Atlantic, causing sea ice to melt. As a consequence, more freshwater enters the ocean, reducing the density at its surface, with the result that convection â and thus the thermohaline circulation â is weakened. The effect is a decreased northward heat transport, ultimately causing an increase in ice formation. Such interactions between ocean and sea ice are important in understanding natural climate variability, especially on the time scale of decades.
Yet sea ice also plays an important role in conn...
Table of contents
- CLIMATE CHANGE: The Point of no Return
- Contents
- Editorâs Foreword
- Authorâs Foreword
- Part 1: The Scientific Principles
- 1 The Climate and the Earth System
- 2 The Greenhouse Effect
- 3 Climate Variability and Prediction
- 4 Climate Modeling
- Part 2: The Climate of the 20th and 21st Centuries
- 5 Manâs Influence on the Climate
- 6 Climate Change Scenarios for the Future
- Part 3: Strategies for the Future
- 7 The Public Discourse
- 8 What must be done?
- Glossary
- Bibliography