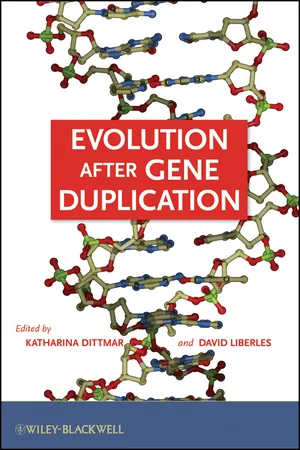
This is a test
- English
- ePUB (mobile friendly)
- Available on iOS & Android
eBook - ePub
Evolution after Gene Duplication
Book details
Book preview
Table of contents
Citations
About This Book
Gene duplication has long been believed to have played a major role in the rise of biological novelty through evolution of new function and gene expression patterns. The first book to examine gene duplication across all levels of biological organization, Evolution after Gene Duplication presents a comprehensive picture of the mechanistic process by which gene duplication may have played a role in generating biodiversity.
Key Features:
- Explores comparative genomics, genome evolution studies and analysis of multi-gene families such as Hox, globins, olfactory receptors and MHC (immune system)
- A complete post-genome treatment of the topic originally covered by Ohno's 1970 classic, this volumeextends coverage to include the fate of associatedregulatory pathways
- Taps the significant increase in multi-gene family data that has resulted from comparative genomics
- Comprehensive coverage that includes opposing theoretical viewpoints, comparative genomics data, theoretical and empirical evidence and the role of bioinformatics in the study of gene duplication
This up-to-date overview of theory and mathematical models along with practical examples is suitable for scientists across various levels of biology as well as instructors and graduate students.
Frequently asked questions
At the moment all of our mobile-responsive ePub books are available to download via the app. Most of our PDFs are also available to download and we're working on making the final remaining ones downloadable now. Learn more here.
Both plans give you full access to the library and all of Perlegoâs features. The only differences are the price and subscription period: With the annual plan youâll save around 30% compared to 12 months on the monthly plan.
We are an online textbook subscription service, where you can get access to an entire online library for less than the price of a single book per month. With over 1 million books across 1000+ topics, weâve got you covered! Learn more here.
Look out for the read-aloud symbol on your next book to see if you can listen to it. The read-aloud tool reads text aloud for you, highlighting the text as it is being read. You can pause it, speed it up and slow it down. Learn more here.
Yes, you can access Evolution after Gene Duplication by Katharina Dittmar, David Liberles in PDF and/or ePUB format, as well as other popular books in Biological Sciences & Genetics & Genomics. We have over one million books available in our catalogue for you to explore.
Information
Chapter 1
Understanding Gene Duplication Through Biochemistry and Population Genetics
1.1 Introduction
Gene duplication has emerged as an important process supporting the functional diversification of genes. Since publication of the seminal book Evolution by Gene Duplication by Ohno (1970), the hypothesis regarding the importance of gene duplication in the generation of evolutionary novelty has steadily gained support as we have entered the genome-sequencing era. It is through the link to functional biology that an ultimate understanding of the preservation and diversification of duplicate genes will be accomplished.
Genes can diverge in function through accumulation (fixation) of coding sequence changes, which may influence binding interactions and/or catalysis, through the evolution of splice variants, and through spatial, temporal, and concentration-level changes in the expression of the protein product. Governing these processes is an interplay among mutational opportunity, population dynamics, protein biochemistry, and systems and organismal biology. This interplay is described systematically in this chapter.
1.2 Systems Biology and Higher-Level Organization
At the level of biological systems, two early but still relevant views suggested a role for gene duplication in constructing pathways. These views are both dependent on a new function emerging in one of the duplicates, but differ in the manner in which it occurs. One view, patchwork evolution, involved a conservation of catalytic activity coupled with the evolution of a new substrate after duplication (Jensen, 1976). An alternative view, retrograde evolution, suggested that pathways are built up backward, with product becoming substrate based on recognition of the transition state in the active site, with the evolution of a new catalytic activity to generate the substrate for the downstream reaction after duplication (Horowitz, 1945). In a systematic analysis in Escherichia coli, Light and Kraulis found some evidence for the retrograde evolution model, but found the patchwork model to be much more common, possibly because it is easier to gain new binding specificity than to evolve a new catalytic activity (Light and Kraulis, 2004). Relatedly, it has been suggested that (also in bacteria) there are secondary (moonlighting) functions where enzymes with a given catalytic activity carry it out on multiple substrates with different specificities (Copley, 2003). This nature of enzymatic activities might generally lead to quick differential optimization after duplication, especially easily if maintained with different specificities in different alleles by balancing selection before duplication. Further (as discussed in detail below), specificity is chemically and evolutionarily difficult to attain, and nonspecific binding activities may arise easily when there is no selective pressure against them. Whereas selective pressures are ultimately at the systems level, divergence occurs gene by gene and mutation by mutation. This process will be dissected.
1.3 Mutational Dynamics and Substitutions
Both intramolecular and intermolecular coevolution of sites affects the probability of fixation of any individual mutation, where genetic background (the sequence at genetically interacting positions) determines the phenotype of any given mutation. The evolutionary accessibility of different mutations from a given genetic background is therefore dictated partly by the mutation rate and the frequency of multiple segregating mutations as well as the population size as a dictator of strength of selection. The same evolutionary properties affect both intramolecular and intermolecular interaction, only with differing degrees of sensitivity to mutation, due to the entropic differences between the two types of interresidue interaction. For these entropic reasons, it is easier to knock out a binding interaction than to knock out proper protein folding (although this happens, too) with a single mutation. This is because although there are a greater number of sites that influence proper folding, covalent attachment means that there will also be a greater local effective concentration of intramolecularly interacting residues requiring a lower affinity interaction to generate the same levels of bound state. If one views two residues as interacting or not interacting, the probability of interaction at any given time is dependent on their affinity for each other and how many opportunities they have to interact (their concentration about each other).
So far, we have focused on the coding properties of a gene. Gene expression is another important process that is subject to phenotypic divergence through mutation. The typical gene has approximately 12 transcription factor binding sites [the distribution of this across genomes is not well characterized, and this number is given with an approximation of six to eight base pairs (Harbison et al., 2004; Hughes and Liberles, 2007)]. The specificity of binding typically enables transcription factors to discriminate among many sites with single-base-pair mutations (Lusk and Eisen, 2008). Because of the small size of transcription factorâbinding sites, site loss and de novo site evolution are reasonably common, and this is explored further below. Due to the periodicity of standard B-form DNA of about 10 bp, as well as changes in effective local concentration of transcription factors about each other and about the initiation site, it might be expected that spacing between sites is important in gene regulation, but evidence generated so far seems to downplay the role of these effects (Shultzaberger et al., 2007), leading to a focus on the evolution of the sites themselves.
Splicing is another mechanism by which genes can diverge through mutation. There are two types of splicing, constitutive and alternative, with alternative splicing simply showing a weaker consensus to splicing regulatory sites (Churbanov et al., 2008). Like transcription factorâbinding sites, splicing regulatory sequences are short and potentially subject to turnover. However, because of the lack of redundancy (unlike transcription factorâbinding sites), loss in the absence of duplication may frequently be highly deleterious. It has been shown that alternative splicing itself enables a substitution burst mediated by relaxed selection on and around these regulatory sites (Xing and Lee, 2005). That gene duplication can also enable such a burst of substitution under relaxed selection suggests that gene duplication should enable enhanced rates of alternative transcript generation, and this has indeed now been demonstrated (Jin et al., 2008).
Many other molecular mechanisms can contribute to mutation-driven diversification. A far from exhaustive list would include glycosylation sites, protein splicing, and RNA editingâone only needs to think of the effects of duplication and relaxed selection on any processes generating constraint described in a molecular biology textbook.
Starting with a few examples of several of these molecular processes, we will then link mutational opportunity to evolutionary mechanism and process. The following section includes a series of examples of the fates of duplicate genes. These examples are meant to be illustrative, and we will ultimately address how general the various processes that underlie the examples actually are.
1.4 Evolution of Enzyme Active Centers After Duplication
Mutations in the active center(s) of an enzyme can lead to a change of its substrate or a change in its kinetics. For example, Vick and Gerlt (2007) demonstrate that a single-base-pair change leading to D-to-G substitution in the active center of the monofunctional l-Ala-d/l-Glu epimerase from E. coli introduced the ability to catalyze the o-succinylbenzoate synthase reaction while reducing the level of the original reaction (Figure 1.1). Four additional nucleotide substitutions led to a complete switch of specificity and kinetics to the new reaction. Consistent with the patchwork model discussed earlier, a large number of enzymes in the arginine and lysine synthetic pathways are homologous to each other (Miyazaki et al., 2001).
Figure 1.1 (A) Substitutions in the active center of the L-Ala-D/L-Glu (SP2Q filling residues). (B) Substitution of one nucleotide in codon coding for Asp297 leads to acquiring of o-succinylbenzoate activity. Further substitutions of Arg24 and Ile19 (located on an unresolved loop) lead to a complete switch of specificity of the reaction (PDB ID 1JPD).
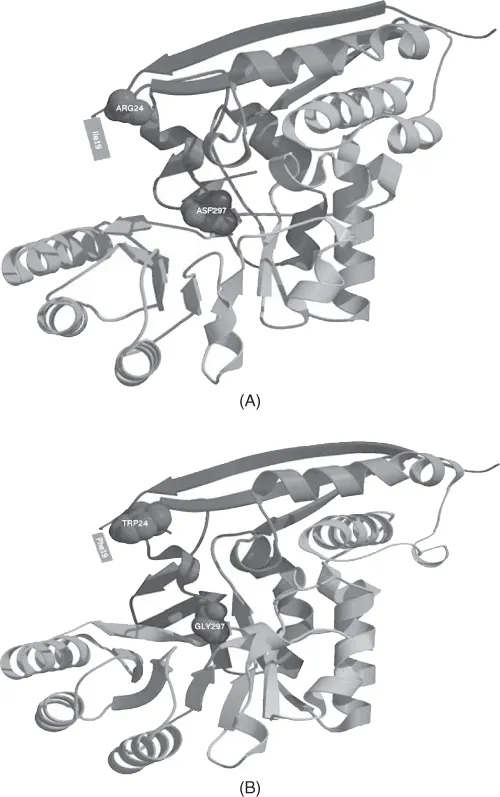
Mutations in the structure surrounding the active center can lead to fine-tuning the active center to different but fundamentally similar substrates. For example, residues in the active centers of Leu-tRNA synthase and Ile-tRNA synthase are mostly conserved; Leu and Ile are very close chemically. There are a number of variable residues that do not directly contact the substrate residue in the active center but, rather, shape the active center, allowing for recognition of the cognate substrate residue. Both tRNA synthases are highly similar on both the sequence and structural levels. Leu- and Ile-tRNA synthases probably arose via gene duplication (Brown and Doolittle, 1995). This demonstrates a shift in substrate specificity following gene duplication.
1.4.1 Change of DNA-Binding Specificity
Homeobox genes are homeodomain-containing transcription factors that are known as principal regulators in the formation of the animal body plan during embryo development. They are often organized in homologous gene clusters such as Hox, ParaHox, and NK (Garcia-FernĂĄndez, 2005). Hox clusters can contain different numbers of genes in different species, where new genes in the clusters arise via duplication and loss in the course of evolution.
It has been shown that the DNA-binding specificity of Hox genes is controlled by a few key positions in the homeodomain. For example, substitution of Gln to Lys in position 50 of the homeodomain alters recognition from TAATCC (recognized by bicoid class hox proteins) to the TAAT(T/G)(A/G) motif recognized by the Antennapedia and Engrailed classes (Hanes and Brent, 1989; Treisman et al., 1989; Percival-Smith et al., 1990). This is shown in Figure 1.2. Similarly, substitutions in positions 3, 6, and 7 of the N-terminus of the homeodomain alter the specificity toward the nucleotide in position 2 of the motif TTATGG â TAATGG (Ekker et al., 1994; Noyes et al., 2008).
Figure 1.2 X-ray and NMR structures of three Hox proteins from Drosophila melanogaster in complex with its DNA recognition site. Mutation in crucial position 50 of the homeodomain switches the specificity to a different DNA binding site. Gln50 in Antennapedia (A) contacts GG on the antisense strand, leading to recognition of the TAATGG core motif, while Lys50 in the bicoid homolog of Antennapedia (B) recognizes TAATCC. The recognition site can be changed mutagenically (Tucker-Kellogg et al., 1997). (C) Ultrabithorax (a tandem duplicate of Antennapedia) has the same DNA-binding motif, but it developed an interaction with the Ebx homeodomain protein mediated primarily by the terminal YPWM motif of Ubx that binds to the hydrophobic pocket in Ebx (Passner et al., 1999). As a result of the interaction, the complex cooperatively recognizes the TGATTTATGG/ATAAATCA motif. The disordered flexible linker (unresolved on the structure) is shown in an extended conformation. (From Baird-Titus et al., 2006.)

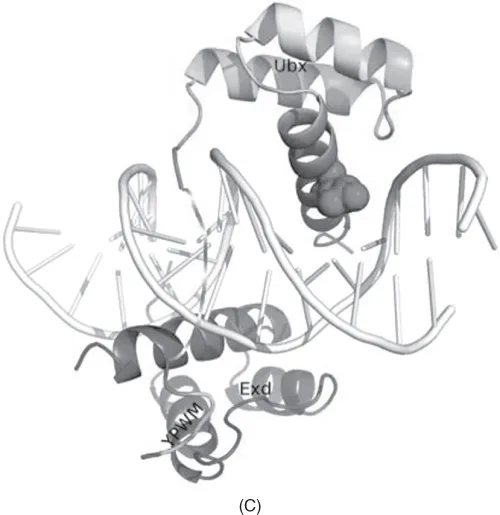
The evolution of homeotic genes in Hox-like clusters demonstrates how gene duplication followed by a single or a few mutations can create new functions that have dramatic effects on the phenotype (in the case of Hox genes, the number of body segments, limbs, etc.). An example of the rearrangement of Hox genes and their regulatory elements is shown in Figure 1.3.
Figure 1.3 HoxA1 and HoxB1 genes in mammals. (a) Wild type. HoxA1 has a fully functioning 3â˛retinoic acid response element (RARE, circle). HoxB1 has both a Hox1 autoregulatory element (ARE, square) and a RARE, with the functionality of the latter severely reduced. (b) Swapping coding regions of the gene in mice produce a normal phenotype. (c) Combing the fully functional ARE and RARE elements around one of the genes and knocking out another also produces a normal phenotype. This is believed to be an ancestral form that preceded WGD and subsequently subfuctionalized so that HoxA1 lost ARE and HoxB1 retained it but deteriorated its RARE. (From Trdvik and Capecchi, 2006.)
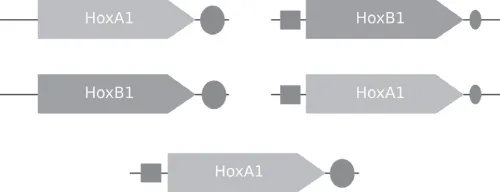
1.4.2 Change of Binding Interface and Interaction Partners
Most proteins do not act alone but, instead, interact with other proteins. This is another mode of potential divergence for duplicated genes. Proteinâprotein interactions are in most cases highly specific and form complex protein interaction networks that execute metabolic functions and make up regulatory, signal transduction, and intercellular circuits. Mutations in the proteinâprot...
Table of contents
- Cover
- Title Page
- Copyright
- Preface
- Contributors
- Chapter 1: Understanding Gene Duplication Through Biochemistry and Population Genetics
- Chapter 2: Functional Divergence of Duplicated Genes
- Chapter 3: Duplicate Retention After Small- and Large-Scale Duplications
- Chapter 4: Gene Dosage and Duplication
- Chapter 5: Myths and Realities of Gene Duplication
- Chapter 6: Evolution After and Before Gene Duplication?
- Chapter 7: Protein Products of Tandem Gene Duplication: A Structural View
- Chapter 8: Statistical Methods for Detecting Functional Divergence of Gene Families
- Chapter 9: Mapping Gene Gains and Losses Among Metazoan Full Genomes Using an Integrated Phylogenetic Framework
- Chapter 10: Reconciling Phylogenetic Trees
- Chapter 11: On the Energy and Material Cost of Gene Duplication
- Chapter 12: Fate of a Duplicate in a Network Context
- Chapter 13: Evolutionary and Functional Aspects of Genetic Redundancy
- Chapter 14: Phylogenomic Approach to the Evolutionary Dynamics of Gene Duplication in Birds
- Chapter 15: Gene and Genome Duplications in Plants
- Chapter 16: Whole Genome Duplications and the Radiation of Vertebrates
- Index