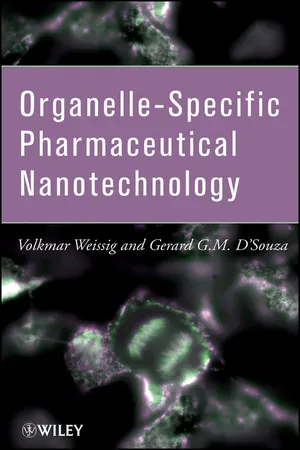
This is a test
- English
- ePUB (mobile friendly)
- Available on iOS & Android
eBook - ePub
Organelle-Specific Pharmaceutical Nanotechnology
Book details
Book preview
Table of contents
Citations
About This Book
This book introduces and discusses the latest in biomedical research--Pharmaceutical Nanotechnology appliedat the sub-cellular level.
Frequently asked questions
At the moment all of our mobile-responsive ePub books are available to download via the app. Most of our PDFs are also available to download and we're working on making the final remaining ones downloadable now. Learn more here.
Both plans give you full access to the library and all of Perlegoâs features. The only differences are the price and subscription period: With the annual plan youâll save around 30% compared to 12 months on the monthly plan.
We are an online textbook subscription service, where you can get access to an entire online library for less than the price of a single book per month. With over 1 million books across 1000+ topics, weâve got you covered! Learn more here.
Look out for the read-aloud symbol on your next book to see if you can listen to it. The read-aloud tool reads text aloud for you, highlighting the text as it is being read. You can pause it, speed it up and slow it down. Learn more here.
Yes, you can access Organelle-Specific Pharmaceutical Nanotechnology by Volkmar Weissig, Gerard G. D'Souza in PDF and/or ePUB format, as well as other popular books in Biological Sciences & Biotechnology. We have over one million books available in our catalogue for you to explore.
Information
CHAPTER 1
An Introduction to Subcellular Nanomedicine: Current Trends and Future Developments
Drug therapy is based largely on the paradigm that an ideal drug will selectively exert a desired pharmacological activity free from negative side effects to modulate either the symptoms or the underlying biochemical cause of a disease to provide a benefit to the patient. In order to have such selective action, the drug molecule should ideally interact with only the disease-associated biochemical pathway but have no activity with respect to any normal biochemical pathway. This principle was famously explored by Paul Ehrlich in his search for agents with selective toxicity toward bacteria. Ehrlichâs work is widely accepted to have given rise to the concept of the ideal drug molecule as a âmagic bullet,â a term that he used for the first time in his Harben Lectures [1]. Finding such selective molecules is relatively easy when there are significant differences between the disease-causing process and normal human biochemical pathways, as in the case of infectious diseases. Not surprisingly, in the decades since Ehrlichâs work infectious diseases have become much easier to treat but it does bear consideration that the lack of activity in nondisease cells is dose dependent and not absolute. Most drugs that are considered to be selectively toxic to invading pathogens are in fact toxic to human cells as well but just at higher doses. However, given that the new challenges in drug therapy lie in the treatment of diseases associated with malfunctions of normal human biochemical pathways in certain tissues, the concept of the magic bullet perhaps needs to be redefined or at least clarified. In the infectious disease example it is essential to understand that the so called magic bullet did not necessarily have to home in on the disease agent but could in fact accumulate to the same level in both host and pathogen cells. Just as long as the agent was toxic only to the pathogen it was considered by many to be a magic bullet. In fact, Ehrlichâs Nobel Prize Lecture from December 11, 1908 speaks of âoutlining the principles of selective toxicityâ rather than selective accumulation. Therefore one could argue that for a molecule to be a magic bullet, it does not have to selectively accumulate at its intended site of action but just that it should not exert its action anywhere but at that intended site of action. This is different from what is now referred to as drug targeting.
The term âtargetingâ is most often meant to imply that the molecule is in some way able to selectively accumulate at an intended site of action and that the selective accumulation is associated with its selective action as a magic bullet. Unfortunately (perhaps due to the widespread use of the noun target to describe a potential molecular site of action), there is often the misconception that a drug that is believed to act at a molecular target (noun) is by default also able to target (verb) or âhome inâ on that target (noun). It would therefore be more appropriate to define a true magic bullet as a drug molecule that is specific in its activity for a molecular target but that is also able to selectively accumulate at this molecular target and exert a selective therapeutic action by virtue of both its specific activity and its selective accumulation. This distinction is important when we consider the daunting challenge of developing magic bullets for diseases like cancer and neurodegenerative diseases like Alzheimerâs, as well as hormone imbalance diseases like diabetes that are becoming more widespread. Unless unique molecular targets found exclusively (or at sufficiently higher levels) in the diseased state and not in normal state are discovered, magic bullets by the traditional definition or the compromise of dose-dependent activity at the site of action may not be feasible. Strategies to effectively control the disposition of drug molecules thus represent an important tool for successful therapy.
At a very basic level, selective accumulation is influenced by bioavailability and subsequent biodistribution. In the context of drug molecules, biodistribution is primarily related to physicochemical properties. Many potent drug candidates exhibit low bioavailability due to their limited water solubility. On the other hand, water-soluble compounds display a very limited ability to cross biological membranes, which essentially can exclude them from the cell interior. To overcome the limitations that a compoundâs physicochemical nature can impose on its potential pharmaceutical application, the process of large-scale screening of chemical libraries has been extended beyond just identifying desired bioactivity. Screening approaches routinely incorporate selection for desirable physicochemical properties that might confer high bioavailability as well. On the down side, this approach leads to many potent molecules being excluded from further development because they arenât true magic bullets; that is, while they may have a potent pharmacological action at a desired molecular target they arenât able to find their way exclusively to that target. There is most certainly a growing list of such molecules that are in essence potential drugs if only a delivery strategy can be devised to get them to their molecular target in the human body. As the search for the perfect magic bullet continues there is a significant effort to improve the action of currently available molecules by using targeted delivery approaches. It is not surprising that the field of drug targeting has grown significantly in the effort to develop better therapy. Based on the experience over the decades since Ehrlich first introduced the magic bullet concept, it seems more reasonable to separate the functions of pharmacological action and selective accumulation into properties desired in a drug molecule and in a delivery system, respectively, rather than the traditional expectation that the drug molecule alone possess both properties.
Generally, drug disposition may be modulated via three broad approaches First, the drug molecule might be modified subtly to change its physicochemical properties without adversely affecting its inherent pharmacological action. This is essentially the intent of medicinal chemistry approaches and the concept of structureâactivity relationship (SAR) studies that have now become standard practice and often arenât even considered a means of achieving targeting. The second approach might be considered to be an extension of the first but is different in that it involves using chemistry to conjugate ligands that are often larger than simple organic functional groups to change the biodistribution of a molecule. Again this approach works as long as the conjugation does not adversely affect the desired pharmacological activity of the molecule. Conjugation using selectively cleavable linkers is an extension of this strategy. The third strategy involves the use of some sort of delivery system or a carrier system and does not involve chemical modifications to the pharmacologically active molecule. Pharmaceutical nanocarriers fall into this category and several such technologies are being developed that are fast becoming applicable to a variety of pharmacologically active molecules.
Pharmaceutical nanocarriers offer what might be viewed as a nonchemical approach to modify the disposition of drug molecules. All chemistry can be performed on the components of the nanocarrier system that can then be loaded with the drug to afford targeted delivery [2â5]. Most pharmaceutical nanocarriers can be modified for some level of targeting to specific tissues if not specific cell types. Long circulating liposomes and nanoparticles are able to passively target areas of leaky vasculature by virtue of the EPR effect and can additionally be modified with antibodies or other targeting ligands to afford cell specific recognition [6â10].
However, despite such advances, the improvement in drug action is not always dramatic. This is likely because many drugs act at molecular targets inside the cells and these molecular targets are often in well-organized subcellular structures inside the mammalian cell.
The interior of a cell is very different from an aqueous buffer solution, in which small drug molecules can freely diffuse and randomly interact with potential cosolutes. In addition to the presence of the cytoskeletal network and various dispersed organelles, the cytoplasm contains a large amount of dissolved macromolecules. The concentration of dissolved macromolecules in the nucleoplasm and cytoplasm of living cells has been determined to be between 50 and 400 g/L [11, 12]. Subsequently, transport or diffusion events in such a crowded solution cannot be expected to be the same as those in buffer solutions. Generally, intracellular diffusion has been characterized as hindered diffusion, reflecting among other factors the high level of molecular crowding [13, 14]. Additionally, the fluid-phase viscosity of the cytoplasm and binding to intracellular components are believed to influence the diffusion of solutes inside a cell [15, 16]. While efforts aimed at thoroughly understanding cellular material properties such as cytoplasmic viscosity are currently underway [17], it is generally accepted that the physicochemical properties of the drug also play a major role in determining the subcellular fate of the drug molecule. Consequently, the ability to predict the influence of various properties of the drug molecule on the likely site of accumulation within the cell could prove to be a powerful tool in drug design to either select molecules with a desired subcellular accumulation or identify molecules that would benefit from subcellular targeting strategies.
There is apparent fractal symmetry between the case of drug delivery to a cell and drug delivery to a molecular target inside a subcellular compartment. The cell could be viewed as being a small, slightly simpler but nonetheless highly organized âbodyâ with âorgansâ (organelles) and âcellsâ (defined structures and molecular arrangements) within these organs. It should therefore stand to reason that controlling drug disposition within the cell might also be necessary for optimal drug action [18â27]. Consequently, the next logical step in the development of targeted nanocarriers would be to extend our control over nanocarrier distribution to the subcellular level as well.
At least two major schemes can be imagined to be useful in the design of nanocarriers with the potential for subcellular targeting: the first based on the inherent predisposition of the nanocarrier for a particular compartment and the second based on attaching subcellular targeting ligands to the surface of nanocarriers to redirect their accumulation to the desired compartment. Essential to the latter of these approaches is the use of a subcellular targeting ligand. Such ligands could be, as in the case of leader sequences, derived from normal cellular trafficking processes or, as in the case of triphenyl phosphonium, based on observations of a predisposition of an organic compound for subcellular compartments. The availability of a wide range of subcellular stains is proof enough that there are several molecules with an inherent ability to accumulate in a particular subcellular compartment.
Based on the intracellular distribution of a large variety of fluorophores, a quantitative structureâactivity relationship (QSAR) model for predicting cellular uptake and intracellular distribution of low molecular weight compounds has been proposed [28]. This QSAR approach was recently applied to identify potential common chemical features of molecules that are known to selectively accumulate at or inside mammalian mitochondria within living cells [29]. The QSAR approach has additionally proved useful for the modeling of cationic transfection lipids [30] and could therefore be applicable to predicting the subcellular disposition of a potential therapeutic molecule and even to design molecules with a desired subcellular affinity for the development of subcellular targeting approaches.
Approaches to nanocarrier-mediated subcellular delivery are based on the principle that the subcellular destination of a drug is the same as that of the nanocarrier. Nanocarriers by virtue of their particulate nature are believed to be subject to endocytic cell entry mechanisms and subsequent endolysosomal processing. As such, nanocarriers could be considered to be ideally suited for delivering bioactive molecules to the endolysosomal system. Indeed, directing nanomedicine complexes to the endolysosomal system has increasingly gained attention, as pathological conditions associated with endosomes and lysosomes could potentially benefit from therapies targeting these pathways [31â34]. Although endocytosis is a common mechanism that almost all cells possess for the internalization of macromolecules, a wide array of such vesicular internalization mechanisms exist [31]. For example, nanoscale drug carrier systems taken up by clathrin-dependent receptor-mediated endocytosis (RME) are most likely to undergo lysosomal degradation, while clathrin-independent RME may lead to endosomal accumulation [31]. Consequently, the type of targeting moiety displayed by the nanocarrier system will determine whether the carrier delivers its cargo to either endosomes or lysosomes.
Well-characterized endocytic targeting moieties potentially useful for nanocarrier-mediated drug delivery are folic acid, low-density lipoprotein, cholera toxin B, mannose-6-phosphate, transferrin, riboflavin, the tripeptide RGD, ICAM-1 antibody, and nicotinic acid, as recently reviewed by Bareford and Swaan [31]. The cellular internalization mechanisms utilized by these ligands involve clathrin-dependent RME, caveolin-assisted endocytosis, lipid raft associated endocytosis, and cell adhesion molecule (CAM) directed cellular uptake [31].
In addition to several approaches to exploiting the inherent tendency of nanoparticles to accumulate in the endolysosomal compartment for possible therapeutic purposes, there is a growing body of work that suggests the feasibility of modifying nanocarriers to redirect delivery of their cargo to other subcellular compartments as well. Liposomes modified with mitochondriotropic ligands have been shown to improve the efficacy of an anticancer drug both in vitro and in vivo [35]. AuNPs have already been targeted to the nucleus using the adenoviral nuclear localization signal (NLS) and integrin binding domain [36]. Such an approach has been reported to be useful in the development of probes for cell tracking by surface-enhanced Raman scattering [37]. Modification with a leader sequence peptide has also been applied to creating delivery systems for mitochondria. A mitochondrial leader peptide (MLP), derived from the nucleocytosol expressed but mitochondria localized ornithine transcarbamylase, has been reported to render polyethylene imine (PEI) mitochondriotropic and represents a potential approach for mitochondrial DNA delivery [38].
It is interesting to note that the examples discussed above share a common assumption. Nanocarriers are assumed to have a predisposition for the endolysosomal pathway by virt...
Table of contents
- Cover
- Title page
- Copyright page
- PREFACE
- CONTRIBUTORS
- CHAPTER 1 An Introduction to Subcellular Nanomedicine: Current Trends and Future Developments
- CHAPTER 2 Delivery of Nanosensors to Measure the Intracellular Environment
- CHAPTER 3 Cytoplasmic Diffusion of Dendrimers and Dendriplexes
- CHAPTER 4 Endocytosis and Intracellular Trafficking of Quantum DotâLigand Bioconjugates
- CHAPTER 5 Synthesis of Metal Nanoparticle-Based Intracellular Biosensors and Therapeutic Agents
- CHAPTER 6 Subcellular Fate of Nanodelivery Systems
- CHAPTER 7 Intracellular Fate of Plasmid DNA Polyplexes
- CHAPTER 8 Intracellular Trafficking of Membrane Receptor-Mediated Uptake of Carbon Nanotubes
- CHAPTER 9 Real-Time Particle Tracking for Studying Intracellular Transport of Nanotherapeutics
- CHAPTER 10 Tracking Intracellular Polymer Localization Via Fluorescence Microscopy
- CHAPTER 11 Can QSAR Models Describing Small-Molecule Xenobiotics Give Useful Tips for Predicting Uptake and Localization of Nanoparticles in Living Cells? And If Not, Why Not?
- CHAPTER 12 Self-Unpacking Gene Delivery Scaffolds
- CHAPTER 13 Cellular Trafficking of Dendrimers
- CHAPTER 14 Endolysosomolytically Active pH-Sensitive Polymeric Nanotechnology
- CHAPTER 15 Uptake and Intracellular Dynamics of Proteins Internalized by Cell-Penetrating Peptides
- CHAPTER 16 Cargo Transport by Teams of Molecular Motors: Basic Mechanisms for Intracellular Drug Delivery
- CHAPTER 17 The Potential of Photochemical Internalization (PCI) for the Cytosolic Delivery of Nanomedicines
- CHAPTER 18 Peptide-Based Nanocarriers for Intracellular Delivery of Biologically Active Proteins
- CHAPTER 19 Organelle-Specific Pharmaceutical Nanotechnology: Active Cellular Transport of Submicro- and Nanoscale Particles
- CHAPTER 20 Subcellular Targeting of Virus-Envelope-Coated Nanoparticles
- CHAPTER 21 Mitochondria-Targeted Pharmaceutical Nanocarriers
- CHAPTER 22 Cell-Penetrating Peptides for Cytosolic Delivery of Biomacromolecules
- CHAPTER 23 Therapeutic Nano-object Delivery to Subdomains of Cardiac Myocytes
- CHAPTER 24 Design Parameters Modulating Intracellular Drug Delivery: Anchoring to Specific Cellular Epitopes, Carrier Geometry, and Use of Auxiliary Pharmacological Agents
- CHAPTER 25 Uptake Pathways Dependent Intracellular Trafficking of DNA Carrying Nanodelivery Systems
- CHAPTER 26 Cellular Interactions of Plasmon-Resonant Gold Nanorods
- CHAPTER 27 Quantum Dot Labeling for Assessment of Intracellular Trafficking of Therapeutically Active Molecules
- Index
- Color Plates