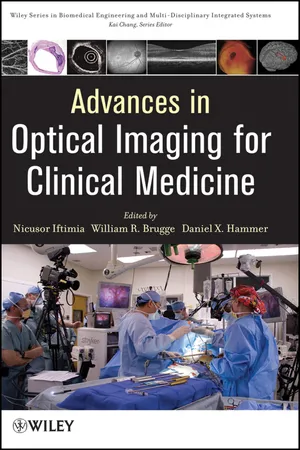
eBook - ePub
Advances in Optical Imaging for Clinical Medicine
Nicusor Iftimia, William R. Brugge, Daniel X. Hammer, Nicusor Iftimia, William R. Brugge, Daniel X. Hammer
This is a test
- English
- ePUB (mobile friendly)
- Available on iOS & Android
eBook - ePub
Advances in Optical Imaging for Clinical Medicine
Nicusor Iftimia, William R. Brugge, Daniel X. Hammer, Nicusor Iftimia, William R. Brugge, Daniel X. Hammer
Book details
Book preview
Table of contents
Citations
About This Book
This book provides students, teachers, researchers and clinicians with a strong and established source of information on advanced optical technologies that show real promise of being translated to clinical use.
Frequently asked questions
At the moment all of our mobile-responsive ePub books are available to download via the app. Most of our PDFs are also available to download and we're working on making the final remaining ones downloadable now. Learn more here.
Both plans give you full access to the library and all of Perlegoās features. The only differences are the price and subscription period: With the annual plan youāll save around 30% compared to 12 months on the monthly plan.
We are an online textbook subscription service, where you can get access to an entire online library for less than the price of a single book per month. With over 1 million books across 1000+ topics, weāve got you covered! Learn more here.
Look out for the read-aloud symbol on your next book to see if you can listen to it. The read-aloud tool reads text aloud for you, highlighting the text as it is being read. You can pause it, speed it up and slow it down. Learn more here.
Yes, you can access Advances in Optical Imaging for Clinical Medicine by Nicusor Iftimia, William R. Brugge, Daniel X. Hammer, Nicusor Iftimia, William R. Brugge, Daniel X. Hammer in PDF and/or ePUB format, as well as other popular books in Biological Sciences & Biotechnology. We have over one million books available in our catalogue for you to explore.
Information
Chapter 1
Introduction to optical imaging in clinical medicine
1.1 Brief history of optical imaging
Throughout history, as soon as a piece of optical apparatus was invented, human beings have used it to gaze both outward and inward. The refractive power of simple lenses made from quartz dates back to antiquity. The modern refractive telescope was invented in the Netherlands by Lippershey in 1608 and refined and used widely by Galileo in Italy during the Renaissance to discover the satellites of Jupiter, among other extraterrestrial objects. The modern microscope was also invented in the Netherlands several years earlier, in 1595, by the same lens and eyeglass makers (Lippershey, Sacharias Jansen, and his son, Zacharias). Soon thereafter it was used to probe the microarchitecture of the human cell. Both telescopes and microscopes have evolved considerably over the years, guided by the elegantly simple fundamental physical laws that govern optical image formation by refraction proffered mathematically by Willebrord Snellius in 1621. Indeed, the principles of optics can simultaneously seem exceedingly simple and irreducibly complex. The early work in the Renaissance on the theory of diffraction and dispersion was led by Descartes, Huygens, and Newton, and was followed by the famous double-slit experiment of Young, which was subsequently supported by theory and calculations by Fresnel. The end of the nineteenth century saw the application of interferometry (Michelson) followed by the rise of quantum optics in the twentieth century. These scientific giants and their work provide the framework upon which all the fields and applications discussed in this book are built.
1.2 Introduction to medical imaging
The dawn of the modern era of medical diagnosis can be traced to 1896, when Wilhelm Roentgen captured the first x-ray image, that of his wife's hand (1). The development of radiology grew rapidly after that. Many noninvasive radiologic methodologies have been invented and applied successfully in clinical medicine and other areas of biomedical research. For the first 50 years of radiology, the primary examination involved creating an image by focusing x-rays through the body part of interest and directing them onto a single piece of film inside a special cassette. Later, modern x-ray techniques have been developed to significantly improve both the spatial resolution and the contrast detail. This improved image quality allows the diagnosis of smaller areas of pathology than could be detected with older technology. The next development involved the use of fluorescent screens and special glasses so that the physician could see x-ray images in real time. This caused the physician to stare directly into the x-ray beam, creating unwanted exposure to radiation. A major development along the way was the application of pharmaceutical contrast agents (dyes) to help visualize, for the first time, blood vessels, the digestive and gastrointestinal systems, bile ducts, and the gallbladder. The discovery of the image intensifier in 1955 has also contributed to the further blossoming of x-ray-based technology. Digital imaging techniques were implemented in the 1970s with the first clinical use and acceptance of the computed tomography (CT) scanner, invented by Godfrey Hounsfield (2).
Nuclear medicine (also called radionuclide scanning) also came into play in the 1950s. Nuclear medicine studies require the introduction into the body of very low-level radioactive chemicals. These radionuclides are taken up by the organs in the body and then emit faint radiation signals which are detected using special instrumentation. Imaging techniques that derive contrast from nuclear atoms, such as positron emission tomography (PET) and single-photon emission computed tomography (SPECT), reveal information about the spatiotemporal distribution of a target-specific radiopharmaceutical, which in turn yields information about various physiological processes, such as glucose metabolism and blood volume and flow (3, 4, 5). Magnetic resonance imaging (MRI) provides structural and functional information from concentration, mobility, and chemical bonding of hydrogen (6, 7). All this information is essential for the early detection, diagnosis, and treatment of disease.
In the 1960s the principles of sonar (developed extensively during World War II) were applied to diagnostic imaging. The process involves placing a small device called a transducer against the skin of a patient near the region of interest, such as the kidneys. The transducer produces a stream of inaudible high-frequency sound waves that penetrate the body and bounce off the organs inside. The transducer detects sound waves as they bounce off or echo back from the internal structures and contours of the organs. These waves are received by an ultrasound machine and turned into live pictures through the use of computers and reconstruction software. For example, in ultrasonography, images reveal tissue boundaries (6).
In addition to these traditional imaging modalities, optical imaging began to play a significant role in clinical medicine in early 1960. Optical imaging at both the macroscopic and microscopic levels is used intensively these days by clinicians for diagnosis and treatment. New advances in optics, data acquisition, and image processing made possible the development of novel optical imaging technologies, including diffuse tomography, confocal microscopy, fluorescence microscopy, optical coherence tomography, and multiphoton microscopy, which can be used to image tissue or biological entities with enhanced contrast and resolution (8). Optical imaging technologies are more affordable than conventional radiological technologies and provide both structural and functional information with enhanced resolution. However, the optical techniques still lack sensitivity and specificity for cancer detection. Within the past few years, there has been increased interest in improving the clinical effectiveness of optical imaging by combining two or more optical imaging approaches (or integrating optical imaging technologies into traditional imaging modalities) (9, 10, 11).
Emerging optical technologies are now combined with novel exogenous contrast agents, including several types of nanovectors (e.g., nanoparticles, ligands, quantum dots), which can be functionalized with various agents (such as antibodies or peptides) that are highly expressed by cancer receptors (12, 13, 14, 15). These techniques provide improved sensitivity and specificity and make possible in situ labeling of cellular proteins to obtain a clearer understanding of the dynamics of intracellular networks, signal transduction, and cellācell interactions (16, 17, 18, 19, 20). Ultimately, the combination of these new molecular and nanotechnology approaches with new high-resolution microscopic and spectroscopic techniques (e.g., optical coherence tomography, optical fluorescence microscopy, scanning probe microscopy, electron microscopy, and mass spectrometry imaging) can offer molecular resolution, high sensitivity, and a better understanding of the cell's complex āmachineryā in basic research. The resulting accelerated progress in diagnostic medicine could pave the way to more inventive and powerful gene- and pharmacologically based therapies.
1.3 Outline of the book
The desire for powerful optical diagnostic modalities has motivated the development of powerful imaging technologies, image reconstruction procedures, three-dimensional rendering, and data segmentation algorithms. In particular, the overwhelming problem of light scattering that occurs when optical radiation propagates through tissue and severely limits the ability to image internal structure is discussed. The broad range of methods that have been proposed during the past decade or so to improve imaging performance are presented. The relative merits and limitations of the various experimental methods are discussed. We consider whether the new advanced approaches will contribute further to the likelihood of successful transition from benchtop to bedside. A brief presentation of each chapter follows.
Chapter 2 outlines traditional clinical imaging modalities and their use in therapy planning and guidance, including ultrasound (US), x-ray imaging, computed tomography (CT), magnetic resonance imaging (MRI), positron emission tomography (PET), and single-photon emission computed tomography (SPECT). Although these imaging technologies provide good structural and functional information, they have inherent safety issues (especially x-ray/CT and those used in nuclear medicineāPET/SPECT), and their resolution is limited. Future advances in medical imaging may be possible by combining these traditional imaging modalities with novel optical, molecular, and nano-imaging approaches. The advantages of these multimodal approaches enable higher-resolution structural and functional imaging and disease detection in its early stage when the therapy success rate is relatively high.
Chapter 3 outlines the current imaging approaches in clinical medicine and is authored by leading clinicians from several prestigious teaching hospitals in the United States. This chapter is organized in four independent sections dealing with ophthalmic imaging, imaging of the gastrointestinal mucosa, cardioimaging, and neuroimaging. Current technological approaches, their limitations, and further needs are presented in detail.
Because the eye is the only transparent organ in the body accessible to in vivo and noninvasive examination, it is often the first and best venue for the application of new optical imaging techniques. Optical coherence tomography (OCT) is a prime example. Before this technique spread rapidly to other fields of inquiry, it was perfected in ophthalmology. The optics of the eye can be limiting in terms of theoretical achievable resolution (i.e., ocular aberrations, numerical aperture, etc.) compared to conventional or confocal microscopy. These limitations have, however, provided opportunities for the development of tools to overcome them (e.g., adaptive optics). Chapter 4 is a monograph of the optical-based imaging modalities for ophthalmic use, including conventional fundus imaging, OCT, and scanning laser ophthalmoscopy, and also new technologies such as adaptive optics and polarization imaging.
Confocal microscopy, invented by Minsky in 1957, is an elegant and simple method for achieving high image contrast by reduction of light scatter from adjacent (lateral and axial) voxels. Chapter 5 focuses on reflectance confocal microscopy (RCM) for skin cancer detection. RCM enables real-time in vivo visualization of nuclear and cellular morphology. The ability to observe nuclear and cellular details clearly sets this imaging modality apart from other noninvasive imaging technologies, such as MRI, OCT, and high-frequency ultrasound. The lateral resolution of RCM, typically 0.2 to 1.0\ μm, enables tissue imaging with a resolution comparable to that of high-magnification histology. As a result, RCM is being developed as a bedside tool for the diagnosis of melanoma and nonmelanoma skin cancers. The development and testing of advanced RCM instrumentation by the Memorial SloanāKettering cancer microscopy group is discussed in detail in this chapter.
Chapter 6 is devoted to the clinical applications of OCT in gastroenterology, including the esophagus, stomach, colon, duodenum, and the pancreatic and biliary ducts. OCT is an attractive tool for the interrogation of gastrointestinal tissue because it rapidly acquires optical sections at resolutions comparable to those of architectural histology, is a noncontact imaging modality, does not require a transducing medium, relies on endogenous contrast, and unlike the collection of forceps biopsies, is nonexcisional. Traditional assessment of gastrointestinal tissues is typically performed by endoscopy with accompanying forceps biopsy in organs with a larger-diameter lumen (esophagus and colon) or with brush cytology in the pancreatic or biliary ducts. OCT has shown promise for targeting premalignant mucosal lesions, grading and staging cancer progression, and reducing the risks and sampling error associated with biopsy acquisition. Although biopsy has traditionally been considered the gold standard for the diagnosis of gastrointestinal pathology, in many cases this assessment suffers greatly from sampling errors, with only a small percentage of the involved tissue being imaged. This is especially evident in cases where the disease may be focally distributed. Recent use of high-speed Fourier-domain OCT in the gastrointestinal tract has made significant strides toward comprehensive imaging, which may help to reduce the sampling error associated with the current assessment.
The most recent advances in confocal endomicroscopy for gastrointestinal (GI) cancer diagnosis are presented in Chapter 7. Confocal endomicroscopy is a rapidly emerging optical imaging modality that is currently being translated to routine clinical use. Advances in miniaturization have led to the development of numerous fiber opticābased confocal microscopes which may be deployed through the instrument channel of a conventional endoscope, or permanently packaged within the tip of a custom endoscope. Confocal endomicroscopes can enable real-time, high-resolution, three-dimensional visualization of epithelial tissues for improved early detection of disease and for image-guided interventions such as physical biopsies and endoscopic mucosal resection. Current research effort has focused on overcoming the limitations of confocal microscopy. For example, its limited field of view is now overcome by real-time generation of a mosaic of stitched images. The application of confocal microscopy for the interrogation of epithelial surfaces in the GI tract presents unique design challenges. Therefore, this chapter focuses on clinical motivation, challenges, design parameters, and other fundamental aspects of GI endomicroscopy. Also discussed are the latest efforts to develop miniature confocal microscopes that are compatible with those of GI endoscopy.
Chapter 8 presents recent progress in minimally invasive approaches in interventional cardiology (IC). The diagnosis of vulnerable plaques occupies an important part of this chapter. The structure of these plaques (i.e., hard core, fluid-filled lesions accessible only with depth-resolved techniques) makes them the most difficult to diagnose, yet they seem to be responsible for most deleterious coronary events. Identification and visualization of high-risk plaques are key to designing customized therapeutic approaches. The ultimate goal is to accurately categorize high-risk patients, target therapy to appropriate areas of vulnerable plaque, and thus prevent or reduce the probability of adverse events. A number of minimally invasive imaging modalities currently in use are presented, including intravascular ultrasound (IVUS) and angioscopy. Also presented are promising new investigational methodologies, including OCT, intracoronary thermography, near-infrared spectroscopy, and intracoronary MRI. OCT in particular uniquely enables excellent resolution of coronary architecture and precise characterization of plaque morphology.
An overview of time-resolved (lifetime) laser-induced fluorescence spectroscopy (TR-LIFS) is presented in Chapter 9. TR-LIFS instrumentation, methodologies for in vivo characterization, and diagnosis of biological systems are presented in detail. Emphasis is placed on the translational research potential of TR-LIFS and on determining whether intrinsic fluorescence signals can be used to provide useful contrast for the diagnosis of high-risk atherosclerotic plaque and brain tumors intraoperatively.
Chapter 10 outlines the use of near-infrared spectroscopy (NIRS) for noninvasive monitoring of brain hemodynamics and oxidative metabolism (i.e., oxygenation status). This technology is capable of monitoring cerebral activity in response to various stimuli (motor, visual, and cognitive) and therefore is currently being used to study functional processes in the brain, to diagnose mental diseases, and more precisely, to localize brain injuries. Clinical demonstration and widespread use of this technology is expected to increase in the coming years because of its promise for functional imaging. In particular, major breakthroughs are expected in cutting-edge techniques such as time-domain functional near-infrared spectroscopy. Current research systems employ low-power pulsed diode lasers and complex, efficient detection instrumentation. Future prototype clinical instruments will achieve higher signal/noise ratios through the use of ultrafast (picoseconds), high-power (>1 W) broadband fiber laser and miniaturized, sensitive, and fast photonic crystal devices combined with high-throughput photodetection electronics.
Chapter 11 describes the application of NIRS to mammography. In optical mammography, diagnosis is based on the detection of local differential concentrations of endogenous absorbers and/or scatterers between normal and diseased breast tissue. Various implementation approaches in conjugation with MR imaging are discussed in detail. The prevalence and implication of age, hormonal status, weight, and demographic factors on complex structural changes in the breast are discussed as well. Such intra- and interpatient variations affect the effectiveness of these imaging modalities adversely and to date have restricted wide clinical use to a specific demographic or time window. As clinical optical mammography matures, its potential impact on cancer management ...
Table of contents
- Cover
- Series Page
- Title Page
- Copyright
- Dedication Page
- Contributors
- Preface
- Chapter 1: Introduction to optical imaging in clinical medicine
- Chapter 2: Traditional Imaging Modalities in Clinical Medicine
- Chapter 3: Current imaging approaches and further imaging needs in clinical medicine: A clinician's perspective
- Chapter 4: Advances in Retinal Imaging
- Chapter 5: Confocal Microscopy of Skin Cancers
- Chapter 6: High-resolution optical coherence tomography imaging in gastroenterology
- Chapter 7: High-resolution confocal endomicroscopy for gastrointestinal cancer detection
- Chapter 8: High-resolution Optical Imaging in Interventional Cardiology
- Chapter 9: Fluorescence Lifetime Spectroscopy in Cardio- and Neuroimaging
- Chapter 10: Advanced Optical Methods for Functional Brain Imaging: Time-Domain Functional Near-Infrared Spectroscopy
- Chapter 11: Advances in Optical Mammography
- Chapter 12: Photoacoustic Tomography
- Chapter 13: Optical Imaging and Measurement of Angiogenesis
- Chapter 14: High-resolution Phase-Contrast Optical Coherence Tomography for Functional Biomedical Imaging
- Chapter 15: Polarization imaging
- Chapter 16: Nanotechnology Approaches to Contrast Enhancement in Optical Imaging and Disease-Targeted Therapy
- Chapter 17: Molecular probes for optical contrast enhancement of gastrointestinal cancers
- Index