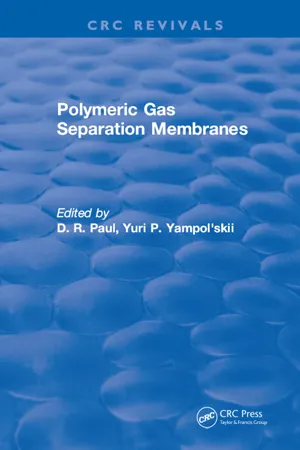
This is a test
- 635 pages
- English
- ePUB (mobile friendly)
- Available on iOS & Android
eBook - ePub
Polymeric Gas Separation Membranes
Book details
Book preview
Table of contents
Citations
About This Book
Polymeric Gas Separation Membranes is an outstanding reference devoted to discussing the separation of gases by membranes. An international team of contributors examines the latest findings of membrane science and practical applications and explores the complete spectrum of relevant topics from fundamentals of gas sorption and diffusion in polymers to vapor separation from air. They also compare membrane processes with other separation technologies. This essential book will be valuable to all practitioners and students in membrane science and technology.
Frequently asked questions
At the moment all of our mobile-responsive ePub books are available to download via the app. Most of our PDFs are also available to download and we're working on making the final remaining ones downloadable now. Learn more here.
Both plans give you full access to the library and all of Perlego’s features. The only differences are the price and subscription period: With the annual plan you’ll save around 30% compared to 12 months on the monthly plan.
We are an online textbook subscription service, where you can get access to an entire online library for less than the price of a single book per month. With over 1 million books across 1000+ topics, we’ve got you covered! Learn more here.
Look out for the read-aloud symbol on your next book to see if you can listen to it. The read-aloud tool reads text aloud for you, highlighting the text as it is being read. You can pause it, speed it up and slow it down. Learn more here.
Yes, you can access Polymeric Gas Separation Membranes by D.R. Paul in PDF and/or ePUB format, as well as other popular books in Ciencias físicas & Química. We have over one million books available in our catalogue for you to explore.
Information
Chapter 1
INTRODUCTION AND PERSPECTIVE
TABLE OF CONTENTS
I. | Membranes Broadly Defined |
II. | Historical Survey A. Fundamentals B. Materials C. Membranes |
III. | Applications: Past and Future |
References | |
Reading List: Some Recent Books on Membranes |
This book is a collection of chapters written by different authors to fulfill several goals. In addition to providing a state-of-the-art review of the field of polymeric gas separation membranes, it was conceived as a specialized textbook for students or those beginning to work in the area. It is also intended for the professional who is experienced in some areas of the field but wants to gain broader knowledge. Finally, it is our hope that this book will help guide the future course of research and commercial practice in this field. To achieve these goals, we felt it was necessary to include a balance of fundamentals, current applications, and consideration of future possibilities. The chapters have been arranged to achieve a logical flow that might be useful for students who wish to read from cover to cover; however, each chapter has been designed to stand alone well enough that the professional can read them in any order depending on interests or needs. Naturally this means a certain amount of repetition. This introductory chapter is intended to give some background and perspective to aid understanding of what follows.
I. MEMBRANES BROADLY DEFINED
It seems appropriate to begin the introductory chapter with an analysis of the notion of “what is a membrane?” In doing so, we must bear in mind that polymeric gas separation membranes, which are the subject of this book, are only a particular case of a much wider field of membranes used in different processes and based on various principles of operation.
It is well known that to give a brief but general and complete definition, i.e., without enumerating the examples or features, is more difficult the wider the class of objects to be covered by the definition. As it has been noted in a witty manner:1 any absolute definition (of a membrane) will be, most likely, incorrect; any correct definition will be incomplete. Nevertheless, many attempts have been made to give a definition of membranes.2,3,4,5 The most general one may be the following:2,5
A membrane is a phase or a group of phases that lies between two different phases, which is physically and/or chemically distinctive from both of them and which, due to its properties and the force field applied, is able to control the mass transport between these phases.
This definition does not imply that membranes are two-dimensional or thin objects, although this is true for the vast majority of existing membranes. No indication of the state of aggregation of the membrane is included; i.e., solid, liquid, or even gas (e.g., gas-filled) membranes are possible. It is reasonable to exclude interphase boundaries between phases, which are thermodynamically distinct from the bulk substance of both phases, although very thin objects like mono- or bilayer films or Langmuir-Blodgett films would, certainly, satisfy this definition.
The etymological significance of the term “membrane”, i.e., “that which covers the members of the body”,6 returns us to a time as early as the seventeenth century when membranes were the object of interest for zoologists and physicians. Interestingly, the first mention of membrane science, membranologia, can be traced to the end of the eighteenth century.6 Needless to say, it dealt solely with biological membranes.
Rather than further pursuing attempts to define membranes, it is probably more fruitful to consider their function or purpose in the context of this book. Membrane methods are now used to perform separations for a wide variety of mixtures of increasing diversity and scale. Although specific membrane techniques may differ from each other in terms of what is being separated, the nature of the membrane, the driving force, and the conditions of operation, they have some things in common. In this respect, they differ from traditional methods of separation like distillation, crystallization, adsorption, absorption, etc., which are based on entirely independent principles. Membrane methods enable one to separate very diverse mixtures, beginning from gas mixtures containing the smallest existing molecules like H2 and He to mixtures of particles beyond molecular sizes, such as viruses or bacterial cultures. Gaseous, supercritical, and liquid media, even solids in the form of a suspension, can be separated by the means of membranes. The basis for the separation may stem from differences in molecular or particle size, charge, or affinity for the membrane. Membranes have found a wide application in diverse fields such as medicine, ecology, power engineering, chemical industry, agriculture, etc.
All this implies that membrane methods have some common features that should determine their efficiency when compared with alternative or nonmembrane methods. The same conclusion is suggested by the important role that is played by membranes in living organisms developed during billions of years of evolution. On the other hand, physicochemical phenomena that form the foundations of some membrane and traditional processes (sorption, complex formation, diffusion, etc.) are often the same.
II. HISTORICAL SURVEY
A. Fundamentals
The first scientific observations related to membrane gas separation were made by J. K. Mitchell of Philadelphia, as early a 1831. He observed that balloons made of india rubber (natural rubber) put into gas atmospheres of different composition blew up with different velocities, depending on the nature of the gas: hydrogen filled balloons faster than air, but the “fastest” gas was carbon dioxide.7 Mitchell noted that carbon dioxide was absorbed by rubber film to a larger degree than other gases, and he was led to infer, accordingly, that rubber expanded in volume and, hence, porosity was induced in the solid sample, which provided a way of penetration of CO2 molecules.
At approximately the same time, A. Fick, who was a physiologist, studied gas transport across membranes made of nitrocellulose and formulated what is known today as Fick’s first law. Of course, the significance of Fick’s law is quite general for many scientific fields, but it is interesting that membranes were the media where it was first established.
However, the paper that determined the direction of thought and research for many decades was published in 1866 by Sir Thomas Graham of University College London, the discoverer of Graham’s law of gas effusion and the father of colloid chemistry.8 He repeated Mitchell’s experiments with films of natural rubber and made the first quantitative measurements of the rate of gas permeation. In his experiments, gas permeated through the film into vacuum not into air. Graham established a series of relative permeation rates across the film for a number of gases that is amazingly close to modern estimates of the corresponding properties. He noted that there was no relation between these values and known diffusion coefficients in gases. So the model now known as the “solution-diffusion” mechanism was proposed: “The first absorption of the gas by rubber must depend upon the nature of a gas.” The absorbed gas “comes to evaporate … and reappears as gas on the other side of the membrane.”
Many other important firsts in gas permeation research, or membrane science more generally, can be attributed to Graham. He carried out the first membrane gas separation and obtained oxygen-enriched air containing 46.6% oxygen. He proposed that increasing the pressure of a gas mixture to be separated should be beneficial for obtaining higher fluxes. He observed that changes in the thickness of films affects the flux but not the composition of permeated gas. He noted the effect of temperature on permeation rates. He prepared the first composite membranes and tried to vary deliberately the chemical nature of the membrane material (gutta percha, gelatin, and other films beside natural rubber). The last but not least interesting detail is that in the second part of his paper9 Graham described his experiments on hydrogen permeation across membranes made of platinum, palladium, and other metals and concluded that they as well as rubber films behaved like non-porous septa.
Steadily, information on permeation through nonporous films was accumulating. Thus, Aronstein and Sirks found that not only gases but water vapor as well is able to penetrate through rubber barriers.10 Exner observed gas permeation across liquid soap films.11 These observations on the permeation rates of different gases indicated that the combination of high solubility and diffusivity is the condition for large permeation fluxes. A quantitative form of this claim was given, probably, by von Wroblewski who defined what we now call the permeability coefficient as the flux Q times the ratio l/Δp, where l is the thickness of the membrane, and Δp is the difference between the upstream and downstream pressures.12 He showed also that the permeability coefficient P can be represented as the product of diffusivity and solubility coefficients, although the units used for the latter differed from those that are in use now.
All these early studies referred to steady fluxes across the membrane. Nearly half a century passed until the importance of transient permeation experiments was realized. H. A. Daynes,13 using a recently devised instrument (a heat conductivity detector, or katharometer, the theory of which he had developed), designed a cell that allowed measurement of “time-deflexion curves” that included both the transient and steady-state transport across the rubber film. The first objective of his work was to check what is the chief obstacle to the passage of gas: the surface of the film or the material itself. However, after solving Fick’s second law under appropriate initial and boundary conditions, he showed that the extrapolation of the line obtained, after steady-state conditions are established, to the time axis gives a time lag L or θ that is directly related to the diffusion coefficient: L = l2/6D. Because it was possible to measure the permeability coefficient P from the steady-state part of the same curve, one could calculate the solubility coefficient as the ratio P/D; hence, all three quantities could be obtained from a single experiment. The conclusion made by Daynes actually defined the trend in membrane gas separation studies for many years. He wrote: “It seems clear that the process of diffusion of a gas through a rubber film is determined by two more or less separate processes. Neither of these obeys simple laws. We can hardly expect, therefore, to go very far in our understanding of the problem by studying permeability alone. Measurement must be made simultaneously on the permeability, absorption coefficients, and diffusion-constants, or, as a minimum, any two of these three quantities.”
This method has been abundantly used. In the 1930s and 1940s, R. M. Barrer widely introduced it to experimental practice, so it is often known as the Daynes-Barrer method. In recognition of the major contributions of Barrer to the field of gas permeation, the following definition for the units of the permeability coefficient is widely used:
A great influence on our knowledge of thermodynamic and diffusion properties of polymers was exerted by the introduction of McBain microbalances. This simple instrument made it possible to obtain abundant information, especially for polymer-vapor systems, on solubility coefficients, sorption isotherms, diffusion coefficients, and sorption kinetics.
B. Materials
The history of research on ...
Table of contents
- Cover
- Title Page
- Copyright Page
- Table of Contents
- Chapter 1 Introduction and Perspective
- Chapter 2 Mechanisms and Theories for Sorption and Diffusion of Gases in Polymers
- Chapter 3 Relationships between Structure and Transport Properties for Polymers with Aromatic Backbones
- Chapter 4 Relationship between Structure and Transport Properties for High Free Volume Polymeric Materials
- Chapter 5 Membrane Formation for Gas Separation Processes
- Chapter 6 Facilitated and Active Transport
- Chapter 7 Unusual Membrane Processes: Non-Steady-State Regimes, Nonhomogeneous and Moving Membranes
- Chapter 8 Membrane Separation of Organic Vapors from Gas Streams
- Chapter 9 Industrial Applications of Membranes for Gas Separation in Japan
- Chapter 10 Commercial and Practical Aspects of Gas Separation Membranes
- Chapter 11 Comparison of Membranes with Other Gas Separation Technologies
- Index