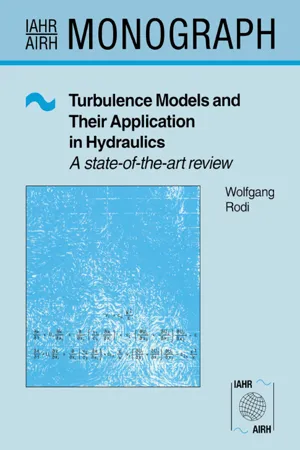
This is a test
- 124 pages
- English
- ePUB (mobile friendly)
- Available on iOS & Android
eBook - ePub
Turbulence Models and Their Application in Hydraulics
Book details
Book preview
Table of contents
Citations
About This Book
This book provides an introduction to the subject of turbulence modelling in a form easy to understand for anybody with a basic background in fluid mechanics, and it summarizes the present state of the art. Individual models are described and examined for the merits and demerits which range from the simple Prandtl mixing length theory to complex second order closure schemes.
Frequently asked questions
At the moment all of our mobile-responsive ePub books are available to download via the app. Most of our PDFs are also available to download and we're working on making the final remaining ones downloadable now. Learn more here.
Both plans give you full access to the library and all of Perlego’s features. The only differences are the price and subscription period: With the annual plan you’ll save around 30% compared to 12 months on the monthly plan.
We are an online textbook subscription service, where you can get access to an entire online library for less than the price of a single book per month. With over 1 million books across 1000+ topics, we’ve got you covered! Learn more here.
Look out for the read-aloud symbol on your next book to see if you can listen to it. The read-aloud tool reads text aloud for you, highlighting the text as it is being read. You can pause it, speed it up and slow it down. Learn more here.
Yes, you can access Turbulence Models and Their Application in Hydraulics by Wolfgang Rodi in PDF and/or ePUB format, as well as other popular books in Technology & Engineering & Civil Engineering. We have over one million books available in our catalogue for you to explore.
Information
1. INTRODUCTION
1.1 The Role of Turbulence Models
In hydraulics, as in other areas of fluid mechanics, the flows of practical relevance are almost always turbulent; this means that the fluid motion is highly random, unsteady and three-dimensional. Due to these complexities, the turbulent motion and the heat and mass-transfer phenomena associated with it are extremely difficult to describe and thus to predict theoretically. Yet, the basic task of hydraulic engineering is that of predicting water-flow phenomena, and, because “predictions” by way of experiments are usually very expensive, calculation methods are in great practical demand.
In spite of all the recent advances in computer technology, turbulent flows cannot at present be calculated with an exact method. The exact equations describing the turbulent motion are known (the Navier-Stokes equations), and numerical procedures are available to solve these equations, but the storage capacity ond speed of present-day computers is still not sufficient to allow a solution for any practically relevant turbulent flow. The reason is that the turbulent motion contains elements which are much smaller than the extent of the flow domain, typically of the order of 10–3 times smaller. To resolve the motion of these elements in a numerical procedure, the mesh size of the numerical grid would have to be even smaller; therefore at least 109 grid points would be necessary to cover the flow domain in three dimensions. Storing the flow variables at so many grid points is still far beyond the capacity of the fast-access memory of present-day computers, and, in addition, the number of arithmetic operations which would be required is so large that the computing time would also be prohibitive. Saffman [1] recently claimed that in about 20 years’ time computers will have been developed with sufficient capacity to solve numerically the exact equations for turbulent flows; for the near future however an exact treatment of turbulence is certainly out of the question.
Until very recently there was not even the glimpse of a hope of ever solving the exact equations for turbulent flows. Since engineers nevertheless needed calculation methods, they took recourse to empirical and semi-empirical methods. Empirical methods simply correlate experimental results and can therefore be used with confidence only for direct interpolation of these results; the Chézy friction law is a typical example. With the aid of dimensional analysis, experimental data were condensed into many useful empirical formulae. However, these can describe only the simplest phenomena of interest and are not suitable for complex geometries; for whenever there are more than just a few parameters determining the problem in question, generally valid empirical correlations are difficult if not impossible to attain. Therefore, at an early stage, another approach was also followed and methods were developed which make use of our theoretical knowledge about fluid-flow phenomena. These methods are based on the conservation laws for mass, momentum and energy and are therefore, at least potentially, of greater general validity than strictly empirical relations. The basic conservation laws are expressed by the exact equations mentioned above, which describe all details of the fluid motion. Because there was (and still is) little hope of solving these equations, and because engineers are in any case not interested in the details of the fluctuating motion, a statistical approach was taken (as first suggested by Osborne Reynolds) and the equations were averaged over a time scale which is long compared with that of the turbulent motion.
The resulting equations describe the distribution of mean velocity, pressure, temperature and species concentration in the flow and thus the quantities of prime interest to the engineer. Unfortunately, the process of averaging has created a new problem : now the equations no longer constitute a closed system since they contain unknown terms representing the transport of mean momentum, heat and mass by the turbulent motion. The system can be closed only with the aid of empirical input, whence the calculation methods based on the averaged flow equations are semi-empirical.
Empirical information can be put into the system of equations in two distinctly different ways. Integral methods, which are suitable mainly for thin shear layers (boundary-layer-type flows), introduce empirical profile shapes so that the originally partial differential equations can be reduced to ordinary ones. Further input is necessary which describes the global effect of turbulence, like the entrainment laws for free shear flows and the friction or energy dissipation laws for wall boundary layers. In contrast, the so-called field methods, which employ the original partial differential equations, require specification of the turbulent transport terms appearing in the equations at each point in the flow. This specification is accomplished by a mathematical model of the turbulent transport processes which is called a “turbulence model”. Therefore, a turbulence model is defined as a set of equations (algebraic or differential) which determine the turbulent transport terms in the mean-flow equations and thus close the system of equations. Turbulence models are based on hypotheses about turbulent processes and require empirical input in the form of constants or functions; they do not simulate the details of the turbulent motion but only the effect of turbulence on the mean-flow behaviour.
Turbulent transport processes are strongly problem-dependent; for example, they depend on geometrical conditions of large and small scale (e.g. wall shape and roughness), on viscous and swirl effects, and on buoyancy. Only the exact but intractable equations form a mathematical model that accurately describes the processes under all possible situations. Turbulence models can only give an approximate description, and, with a particular set of empirical constants, they are valid only for a certain flow or at the most a range of flows.lt is of course desirable in a turbulence model to achieve a good approximation with a single set of constants for a fairly wide range of flows; only then has a field method incorporating the turbulence model real predictive power. A model for which the constants have to be adjusted from flow to flow is in essence little more than a method for interpolating experimental data, similar to the empirical formulae mentioned above. A good turbulence model should however allow extrapolation from the empirical data which entered the model. It is of course important to examine the limits up to which an extrapolation is meaningful.
The most universal turbulence model is not necessarily also the most suitable one for a particular problem. In practical applications,the economy and ease of use of a model are also important factors, and the more universal models are usually more complex and thus require more computing time. Thus, for each problem, the right level of complexity has to be chosen from amongst the available models.
1.2 Scope and Outline of the Review
Starting with Prandtl in 1925, researchers have been increasingly active developing turbulence models during the last 20 years. The first turbulence models, of which the Prandtl-mixing-length hypothesis is the most well-known example, related the turbulent transport terms uniquely to local mean-flow quantities. Even these relatively simple models could, for a long time, be used only to calculate self-similar flows (e.g. far field of jets and wakes) for which the partial differential equations expressing mass and momentum conservation can be reduced to ordinary ones. The partial differential equations governing the more general flow situations could not be solved at that time. Even for the few flows to which they could be applied, the early models lacked universality in that they required different empirical constants for different flows; the desire to remedy this led to the development of more complex models starting in the 1940’s. These models give up the direct, algebraic link between the turbulent transport terms and the mean-flow quantities and employ differential transport equations for turbulence quantities such as the kinetic energy of the turbulent motion. Some of these models are conceptually as advanced as the most complex turbulence models in use today. However, it took another 20 years before these models could actually be applied and tested. In the 1960’s computers became sufficiently powerful and, shortly thereafter, numerical techniques sufficiently well-developed to allow the partial differential equations for the mean flow (and in complex turbulence models) also for turbulence quantities, to be solved for many flow situations. As a consequence, the main effort in developing, and almost all the effort in testing turbulence models has been confined to the last 10 years.
This development and the resulting application of turbulence models was mainly restricted to the area of mechanical and aeronautical engineering. Various reviews are available on this work [2,3,4] , which give a fairly comprehensive picture of the state-of-the-art in these fields. In hydraulic engineering however, the application of turbulence models was, until very recently, restricted to the early, most simple models.
As advanced turbulence models have shown great promise in other fields of engineering, it seems worthwhile (and opportune) for the further development of calculation methods for turbulent-flow problems in hydraulics to review critically the range of available turbulence models with the aim of assessing their suitability for application to hydraulics problems. In particular, it is important to find out how well the various models can cope with the manifold complications present in hydraulics problems, such as irregular geometries, buoyancy and free-surface effects. It is the purpose of this review to present such an assessment and to show, by way of examples, what problems have been and can be tackled with the presently available models.
The number of turbulence models suggested in the literature over the last 70 years is rather large so that not all models can be included in this review. Some of the models suggested have been tested very little (or not at all) and are therefore difficult to assess; others are used no longer because they have been found to perform poorly. The present review concentrates on those models which are still in use and have been tested to a fair degree; further, the models which have already been applied to hydraulic flow problems or are specially geared to them will receive special attention. Assessment of the applications will focus on free surface flows because flows in closed ducts are not peculiar to the field of hydraulics (they are very similar to gas flows occuring in other areas of fluid mechanics and are therefore covered by the reviews mentioned above). Futher, the review concentrates on small-scale (or near-field) issues; for reasons to be explained in detail later, use of the refined models mainly described in this book is not warranted for simulating the large-scale horizontal turbulent transport in natural water bodies. Previous reviews on turbulence models for hydraulic problems can be found in References [5] to [8] . These provide valuable accounts of the state of the art at the time of their appearance, but they are not representative of the present state since significant progress has been achieved in the meantime. In his survey for the 1971 IAHR Congress in Paris, Biesel [8] expressed the hope that one will learn sufficiently quickly from the imperfections of the then existing models in order to soon arrive at models of greater practical value; it is one of the main tasks of this work to examine whether this hope has become true in the meantime.
The present review is restricted to turbulence models describing the local state of turbulence, such as are employed in field methods. The global description of turbulence used in integral methods is not discussed here. Further, the numerical procedure to solve the resulting set of partial differential equations representing the complete mathematical model (mean-flow and turbulence equations) are not dicussed either.Readers interested in numerical procedures in fluid mechanics are referred to the book of Roache [9] .
In the section to follow (Sec. 2.1), the problem of calculating turbulent flows is posed more precisely by introducing and discussing the time-averaged equations governing the mean-flow quantities. The appearance of turbulent transport terms in these equations makes apparent the necessity of introducing turbulence models. Before turning to such models, it is helpful to describe briefly some important features of the turbulence phenomenon, and this is done in Sec. 2.2. In order to review a fairly large number of different turbulence models, a scheme of classification is needed. This scheme is introduced in Sec. 2.3 together with some basic ideas, for example the eddy viscosity concept, common to many models. The heart of this book is the actual review of models in Sections 2.4 to 2.7; the models are discussed in order of increasing complexity. A second major contribution is contained in Chapter 3, where model applications are presented and compared with experimental results, starting with relatively simple problems such as steady channel and jet flows and moving on to increasingly complicated situations, e.g. discharges into stagnant water and into rivers, recirculating and unsteady flows. Chapter 4 closes the review with an assessment of what the available models can achieve and in which areas further research is necessary.
2. TURBULENCE MODELLING
2.1 Mean-Flow Equations and the Problem of Closure
This chapter deals with the equations which govern the distribution of the mean-flow quantities and therefore form the basis of the so-called field methods. The origin of these equations are the conservation laws for mass, momentum, thermal energy and species concentration. For incompressible flows, these laws can be expressed in tensor notation+) as
Mass conservation : continuity equation
(2.1) |
Momentum conservation : Navier-Stokes equations
(2.2) |
Thermal energy / species concentration conservation :
(2.3) |
where Ui is the instantaneous velocity component in the direct...
Table of contents
- Cover
- Half Title
- Title Page
- Copyright Page
- Table of Contents
- PREFACE
- SUMMARY/RESUME
- NOMENCLATURE
- 1. INTRODUCTION
- 2. TURBULENCE MODELLING
- 3. EXAMPLES OF APPLICATIONS TO HYDRAULICS PROBLEMS
- 4. CONCLUSIONS
- APPENDIX A : Introduction to Tensor Notation
- APPENDIX B : The Length Scale Specification Proposed by Bernard et al. [44]
- APPENDIX C : Table 5 : Summary of Turbulence Models and Initial Conditions Used in the Application Examples
- REFERENCES