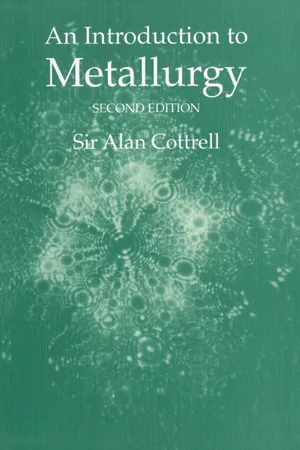
This is a test
- 578 pages
- English
- ePUB (mobile friendly)
- Available on iOS & Android
eBook - ePub
An Introduction to Metallurgy, Second Edition
Book details
Book preview
Table of contents
Citations
About This Book
This classic textbook has been reprinted by The Institute of Materials to provide undergraduates with a broad overview of metallurgy from atomic theory, thermodynamics, reaction kinetics and crystal physics, to elasticity and plasticity.
Frequently asked questions
At the moment all of our mobile-responsive ePub books are available to download via the app. Most of our PDFs are also available to download and we're working on making the final remaining ones downloadable now. Learn more here.
Both plans give you full access to the library and all of Perlegoâs features. The only differences are the price and subscription period: With the annual plan youâll save around 30% compared to 12 months on the monthly plan.
We are an online textbook subscription service, where you can get access to an entire online library for less than the price of a single book per month. With over 1 million books across 1000+ topics, weâve got you covered! Learn more here.
Look out for the read-aloud symbol on your next book to see if you can listen to it. The read-aloud tool reads text aloud for you, highlighting the text as it is being read. You can pause it, speed it up and slow it down. Learn more here.
Yes, you can access An Introduction to Metallurgy, Second Edition by Sir Alan Cottrell in PDF and/or ePUB format, as well as other popular books in Technology & Engineering & Mining Engineering. We have over one million books available in our catalogue for you to explore.
Information
Chapter 1
Prologue
1.1 The art and science of metals
Metallurgy is the art and science of making metals and alloys in forms and with properties suitable for practical use. Most people know it only as an ancient and mysterious art. Certainly it had a place in ancient history, having brought us out of the Stone Age into the Bronze Age and then into the Iron Age. The seemingly miraculous conversion of dull earths into shining metals was the very essence of alchemical mystery; and there was no science of metals to bring rationality and enlightenment into the mediaeval world of secret formulae for tempering metals and blending alloys.
Some of this air of mystery still lingers over metallurgy to this day. No space ship in science fiction is respectable without its own secret âwonder metalâ. This mystery may be a legacy from the past but it is also an unconscious acknowledgement of the many striking achievements of the modern metallurgist in producing new metals and alloys for jet engines, nuclear reactors, electronic circuits and other advanced pieces of engineering. These successes have not been achieved by old dark magic, however, but by the enlightened and logical application of scientific principles. Metallurgy is now a disciplined applied science based on a clear understanding of the structures and properties of metals and alloys. The mystery of the modern wonder metals is due to no more than the simple fact that this science is mostly too new to have filtered down, yet, into the more elementary levels of scientific education.
1.2 Chemical metallurgy
The least remote part of the subject is chemical metallurgy. This deals with all the chemical properties of metals, including the uniting of different metals with one another to form alloys, but a very large part of it is concerned with the oxidation-reduction reactions of metals, for two main practical reasons. First, most metals occur in nature as oxides, sulphides, chlorides, carbonates, etc., and the critical step in converting these ores into metals, i.e. in extraction metallurgy, is a process of chemical reduction. The basic chemical reactions involved are often simple; the scientific challenge in this part of the subject is to achieve these reactions on a massive scale economically. Second, when the finished piece of metal goes into service afterwards and is exposed to the environment, these same chemical reactions tend to occur spontaneously in reverse. The metal thus reverts from the metallic state to the oxidized state, i.e. it rusts or corrodes. The main tasks of the chemical metallurgist are thus to get metals into the metallic state and then to keep them there.
The origins of extraction metallurgy go back into pre-history. The first discoveries must have been made accidentally in camp fires and hearths where stones of easily reducible metallic ores would have been converted to metal by the heat and reducing flames. Copper, lead and tin were amongst the first metals to be made by such a smelting process, over 5000 years ago. At a very early age the alloy bronze, usually about 10 parts of copper to one of tin, was made by smelting mixed ores of the two metals together and was much prized because of its great hardness and because, when melted, it could be cast easily into intricate shapes by letting it solidify in shaped holes in clay or sand moulds. Early brasses were similarly made by smelting mixed copper and zinc ores. The modern method of making alloys by mixing metals was developed later.
Iron ores are also easily reduced but the high melting point of the metal prevented iron from being produced in a liquid form. Instead, a pasty porous mass of sponge iron mixed up with slag (a crude glass containing unreduced oxides and silicates), was produced and this had to be compacted, while hot and soft, by beating or forging it down with hammers, so making something rather like wrought iron. The need for higher temperatures to achieve greater outputs led to the gradual evolution of the early iron-making hearth into the blast furnace, with an air blast directed into the hot zone above the hearth and a tall enclosed stack above, down which the ore and charcoal fuel travelled.
A great advance occurred in the fourteenth century. Temperatures became high enough to produce liquid iron. The blast furnace could then be operated continuously, being periodically âtappedâ to run out the pool of molten iron at the bottom, and this greatly increased its output. The liquid pig iron produced in this way contained about 4 wt per cent dissolved carbon, picked up from the furnace fuel. This carbon greatly lowered the melting point and so made the metal easy to re-melt and cast into moulds. This cast iron was, however, brittle due to the carbon, which forms a brittle iron carbide, and other impurities, and so could not be used for the same purposes as forged sponge iron. The problem of converting pig iron to a ductile form by refining away the carbon was solved by Cort in the eighteenth century with his puddling process for making wrought iron. These two forms of iron, wrought and cast, remained the staple ferrous constructional materials until the later part of the nineteenth century.
The delicate carbon control required to make mild steel (about 0.25 wt per cent carbon) was beyond the scope of the metallurgy of those days. Admittedly, a type of tool steel for swords and cutting tools, which contained about 1 wt per cent carbon and which could be hardened by quenching, red-hot, into cold water, was made from very early times by the cementation process in which forged sponge iron was heated in charcoal; and in 1740 Huntsman made tool steel by melting irons of different carbon contents in a crucible, which was the foundation of the Sheffield cutlery industry. But the discovery that cheap low-carbon steel could be made on a large scale for constructional uses did not come until the mid-nineteenth century, when Bessemer invented his converter process. This was followed a few years later by the open-hearth steelmaking process and the modern age of steel was then begun.
Electricity plays a large part in many modern extraction processes. The decisive step was the Hall-HĂ©roult process for the commercial production of aluminium, announced in 1886. Many other metals such as magnesium, sodium and calcium are also produced electrically, and these metals in turn are now used to produce the âmodernâ metals such as titanium, zirconium, uranium and niobium.
The science of extraction metallurgy has developed rapidly in recent years, with the application of thermodynamics and the theory of reaction kinetics to its problems. The thermodynamics of metallurgical reactions is now well established but there are many opportunities for further advances, both scientific and technological, in the study and control of reaction rates. Many of the newest extraction processes, such as oxygen steel-making, flash roasting, spray refining and the zinc blast furnace process, depend critically on reaction kinetics.
1.3 Mechanical metallurgy
Metallurgy is a branch of a wider subject, known as materials science and engineering, which deals with all materials, e.g. metals, ceramics, glasses, organic plastics and polymers, wood and stone. The reason why metallurgy stands by itself as such a large and self-contained subject is, of course, the outstanding importance of metals as constructional materials. Society as we know it would be quite impossible without metals. The production of metals and metallic goods represents about one-fifth of the gross national product in a modern industrial country.
Metals owe their importance to their unique mechanical properties, the combination of high strength with the ability to change shape plastically (ductility and malleability). This plasticity enables them to be shaped, e.g. into motor car bodies, tin cans and girders, by processes of mechanical working such as pressing, drawing, rolling and forging. Even more important, this same plasticity gives strong metals their extraordinary toughness, the ability to endure all the knocks and shocks of long rough service without breaking or crumbling away.
Mechanical metallurgy deals with all these aspects of the subject; in particular with mechanical working, the testing of mechanical properties, the relations between these properties and engineering design and selection of materials, and the performance of metals in service. It is the oldest part of metallurgy. The earliest known metals, copper, silver and gold, were found native, as metallic nuggets. Meteorites were a source of iron-nickel alloys. All these naturally occurring metals are malleable and, from very early days, they were shaped into ornaments, tools and weapons by hammering. The forging of metals became widely established once extraction metallurgy began to provide copper, bronze, sponge iron and other metals in larger amounts. The Romans made extensive use of lead sheet and pipe in water supply systems. Coining, the indenting of a design on a metal surface with a punch and die, developed at an early age. The advantages of mechanical working at various temperatures were also realized; cold working, because it increased the hardness and strength of metals such as copper and iron; hot working, particularly of sponge iron, because metals proved to be much softer and more malleable at high temperatures and also because they could be joined by pressure welding if hammered together while hot.
The mechanical working of metals remained a largely hand-craft industry, typified by the blacksmithâs forge, for many centuries. The need for larger forgings and the advent of water power eventually led to the power-operated hammer and the forging press. A major development was the rolling mill, which first came into wide use in the eighteenth century. Other processes, such as drawing, machining and extrusion, also gradually evolved. Many new processes have been developed recently, including the cold-forging of steel, using high-pressure lubricants, and explosive forming in which the metal is driven against a die by the force of an explosion. Hydrostatic forming, in which the metal is worked while under great hydrostatic pressure to prevent cracking, seems likely to open up a whole new phase of mechanical metallurgy by enabling the more brittle metals and alloys to be worked.
The science of mechanical metallurgy consists of three main, closely related parts. First, the basic mechanical properties have to be explained from an atomic theory of metals, analogous to the kinetic theory of gases. Here mechanical metallurgy unites with physical metallurgy. Then, starting from these basic properties, the behaviour of metals in mechanical working operations has to be understood and controlled. The attack on this problem has produced a new branch of applied mechanics, the theory of plasticity. Thirdly, again in terms of basic properties, the mechanical behaviour of metals in service has to be understood and improved, to avoid failures due to plastic collapse, brittle cracking, metal fatigue, etc., and to provide a rational basis for engineering design and the efficient and safe use of materials. This is a very active part of the subject at present.
1.4 Physical metallurgy
Few things in nature seem more inanimate than a piece of metal. The casual observer sees only his own reflection in its bright, still surface and nothing of its world within. This internal world is, however, a place of ceaseless activity. Electrons dash from end to end at immense speeds. The atoms themselves also move and exchange places, even when the metal is completely solid. Changes of temperature can cause the atoms to rearrange themselves suddenly into a radically different pattern of organization. In a quenched steel this can happen in a few micro-seconds, even at temperatures far below room temperature. Plastic deformation occurs through the passage of faults, called dislocations, which move at great speed through the metal and cause large-scale slippages between enormous masses of atoms. The traffic of dislocations can become very dense. Huge traffic jams build up, which bring the dislocations to rest and make the metal hard. When this work-hardened metal is heated (annealing) it rids itself of these dislocations in a wave of reorganization of the entire atomic pattern (recrystallization). Completely new atomic patterns can be produced by alloying and these in turn can be changed by heat-treatment. For example, when an aluminium alloy is rested at room temperature, after quenching, its alloy atoms move through the solid to congregate together in small clusters, like water droplets in a mist, and these clusters make the metal hard by getting in the way of dislocations (precipitation hardening).
The study of all effects such as these belongs to the province of physical metallurgy, the part of the subject that deals with the structures of metals and alloys with the aim of designing and producing those structures that give the best properties. Physical metallurgy has obvious links with mechanical metallurgy; but it also has close links with chemical metallurgy, particularly in connection with the casting of metals, the formation of alloys, corrosion, and the many effects of impurities on the structures and properties of metals and alloys. It is the newest part of metallurgy although the processes of quenching and tempering, work-hardening, annealing and alloying were discovered and used, in a completely empirical way, in ancient times. Imaginative attempts to construct a theory of metals, including the essential idea that solid metals might be crystalline, i.e. have their atoms arranged in orderly patterns, were made in the seventeenth and eighteenth centuries. However, there was no way to put these ideas to experimental test in those days and most scientists chose instead to work in fields such as mechanics, astronomy, electricity and chemistry where progress was easier. So the classical pattern of the history of science developed.
The decisive break-through for physical metallurgy was the development by Sorby, in the second half of the nineteenth century, of the metallographic technique for observing the structures of metals and alloys with a reflecting optical microscope. The great barrier of the surface mirror was at last penetrated, by a process of polishing and chemical etching, to reveal the internal structure beneath. What was then seen was the grain structure of metals, an assemblage of minute interlocked crystals. Great changes in this microstructure were seen to be brought about by alloying, working and heat-treatment. Ideas about the nature of these changes sharpened rapidly once it became possible to put them to the test of observation. At about the same time, the theory of thermodynamics was clarifying the understanding of what happens when different substances are blended together and this provided a scientific basis for the study of alloying. The combination of systematic alloy research with optical microscopy opened most of the doors into physical metallurgy. The effects of carbon in steel could be largely understood, as well as the processes of quench-hardening and tempering; the structures and properties of the older alloys, such as the brasses and bronzes, could be rationalized; and a method was at last available for the systematic development of alloys deliberately designed to have certain properties.
The metallurgical microscope is still the most useful general-purpose instrument available to the physical metallurgist. It could not, of course, give direct proof of the crystalline atomic arrangements in metals, although it left little room for doubt. The direct proof had to wait until the discovery of the x-ray diffraction method, the application of which ushered in a second major phase of physical metallurgy in the 1920s. Single crystals of metals were also grown at about this time and their mechanical properties threw much light on the processes of plastic deformation.
The next big advances were theoretical. By the early 1930s the quantum theory of electrons and atoms had become powerful enough to provide a real theory of the metallic state, which could explain what a metal really is and how it conducts electricity. The forces holding metal atoms together could then be understood and a start was made on the theory of alloys. Corrosion was seen (and demonstrated experimentally) to be as much an electrical process as a chemical one and the mobility of atoms in metals was explained in terms of certain well-defined imperfections in the crystal structure (dislocations and vacancies). Theoretical metallurgy was forced along still further in the years immediately after the second world war by the need to develop metals and alloys that would resist high temperatures for jet engines, or which would resist damaging nuclear radiations in nuclear reactors; and by the demands for special materials to be used in the electrical industry. Still more recently, experiment has taken the lead once more, due to the development of extremely powerful electron microscope and field-ion microscope techniques, which allow the structure of metals to be observed right down to the atomic scale of magnitude. The study of dislocations and atomic structures in metals has now become mainly an experimentalistâs science.
The innumerable advances that have taken place in the basic science of metals in recent years have left the considerable problem of digesting them all and converting them into corresponding advances in the applied science. Nevertheless, we can now see clearly how to design the microstructures of metals and alloys so as to develop the basic properties to best effect. Some of the proposed new microstructures are very different from the traditional ones and there is a great technological challenge to realize these commercially on a large scale. As regards the basic science there are still many areas where fundamental problems remain, particularl...
Table of contents
- Cover
- Half Title
- Title Page
- Copyright Page
- Preface
- Table of Contents
- List of Plates
- Units, Conversion Factors, Physical Constants
- 1 Prologue
- 2 The Atomic Nucleus
- 3 Atomic Structure
- 4 Chemical Bonding
- 5 Heat and Energy
- 6 Entropy and Free Energy
- 7 Free Energies of Metallic Compounds
- 8 Extraction of Metals
- 9 Electrochemical Extraction and Refining Processes
- 10 Extraction of Reactive and Refractory Metals
- 11 Iron and Steel Making
- 12 Kinetics of Metallurgical Reactions
- 13 Solids, Liquids and Solidification
- 14 Alloys
- 15 The Phase Diagram
- 16 Ternary Phase Diagrams
- 17 Metal CrystalsâI Periodicity
- 18 Metal CrystalsâII Directionality
- 19 Metal CrystalsâIII Energies and Processes
- 20 Heat-treatment of Alloys
- 21 Mechanical Properties
- 22 Plastic Working
- 23 Oxidation and Corrosion
- 24 Electronic Structure and Properties
- 25 Properties and Uses
- Index