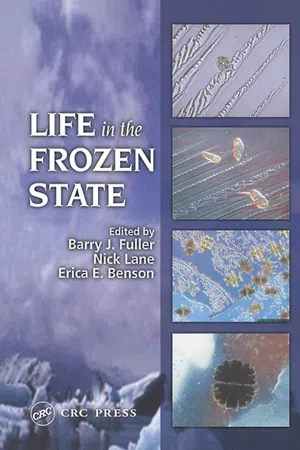
This is a test
- 704 pages
- English
- ePUB (mobile friendly)
- Available on iOS & Android
eBook - ePub
Life in the Frozen State
Book details
Book preview
Table of contents
Citations
About This Book
While it is barely 50 years since the first reliable reports of the recovery of living cells frozen to cryogenic temperatures, there has been tremendous growth in the use of cryobiology in medicine, agriculture, horticulture, forestry, and the conservation of endangered or economically important species.As the first major text on cryobiolog
Frequently asked questions
At the moment all of our mobile-responsive ePub books are available to download via the app. Most of our PDFs are also available to download and we're working on making the final remaining ones downloadable now. Learn more here.
Both plans give you full access to the library and all of Perlegoâs features. The only differences are the price and subscription period: With the annual plan youâll save around 30% compared to 12 months on the monthly plan.
We are an online textbook subscription service, where you can get access to an entire online library for less than the price of a single book per month. With over 1 million books across 1000+ topics, weâve got you covered! Learn more here.
Look out for the read-aloud symbol on your next book to see if you can listen to it. The read-aloud tool reads text aloud for you, highlighting the text as it is being read. You can pause it, speed it up and slow it down. Learn more here.
Yes, you can access Life in the Frozen State by Barry J. Fuller, Nick Lane, Erica E. Benson in PDF and/or ePUB format, as well as other popular books in Medicine & Gynecology, Obstetrics & Midwifery. We have over one million books available in our catalogue for you to explore.
Information
Theme 1
Fundamental Aspects
1 Principles of Cryobiology*
Peter Mazur
1.1 INTRODUCTION
When cells or cellular systems are exposed to the ice formation that accompanies exposure to low subzero temperatures, they are subjected to profound changes in the physical state and chemical properties of their surrounding milieu, and the cells undergo major physical responses to these changes. Liquid water plays a quintessential role in the structure and function of living systems, and the most obvious change accompanying freezing is that the amount of this liquid is progressively reduced and eventually vanishes. The chapter begins by briefly reviewing the structure of both liquid water and ice, some of the ways that liquid water influences the structure of cellular macromolecules, and the nature of water on the surfaces of those macromolecules. The discussion is then extended to cells and their membranes.
Even if liquid water/macromolecule/cell structure interactions were unaffected by freezing, the mere reduction in the concentration of liquid water introduces large osmotic disequilibria to which the cells must respond. We first examine the magnitude of these disequilibria and the magnitude of the volume changes that cells undergo in response to them. We then review the rate at which these responses occur, rates that depend on the permeability of the cell to water and solutes. Finally, this first section relates the phenomenon of permeability to the structure of the cell membrane and to a recently discovered class of transmembrane proteins, the aquaporins.
This background material sets the stage for a discussion of cryobiological principles per se. The discussion begins by pointing out that plots of cell survival vs. cooling rate usually exhibit an inverted Uâfindings that indicate that an injury occurring at high cooling rates has a different genesis than that occurring during slow cooling. The discussion elaborates on the former by pointing out that rapid cooling sets up an osmotic disequilibrium between a cell and its environs and causes cell water to supercool by an amount proportional to the cooling rate and inversely proportional to the permeability of the cell to water. This supercooling cannot be maintained, and eventually supercooled cell water will freeze internally, usually with lethal consequences. The fact that a cell can supercool at all in the presence of external ice means that the plasma membrane is at least initially a barrier to the growth of ice from the exterior to the interior, yet intracellular freezing appears to be dependent on the presence of external ice, and this chapter reviews the three major theories that propose to account for the dependence.
Slowly cooled cells do not undergo intracellular freezing, yet they are generally killed if cooled too slowly and if cryoprotective agents of appropriate type and concentration are not present. Most of the ensuing discussion deals with the colligative theories of both the injury and the protection; that is, theories that propose that injury is associated either with the rise in solute concentration (especially electrolytes) that accompanies ice formation or with its mirror image, the reduction in the unfrozen fraction, and that propose that cryoprotection is related to the ability of certain permeating nontoxic nonelectrolytes like glycerol to reduce the concentrations of damaging solutes and to increase the magnitude of the unfrozen fraction at a given subzero temperature. Some researchers, however, believe that slow-freezing injury is a direct result of the dehydration of cell membranes and that cryoprotection results from the ability of cryoprotective agents to substitute for water or to otherwise protect against the injury that results from the removal or perturbation of the hydration layer on cell membranes.
Finally, freezing is only half the story. To function, a frozen cell must be returned to normal temperatures and must survive the accompanying warming and the thawing of the ice. In the case of slowly frozen cells, the events during the return to ambient temperature are mostly a mirror image of those that occurred during cooling. However, that is not the case with rapidly cooled cells. Commonly, rapidly cooled cells fare substantially better if the subsequent warming is rapid than if it is slow. That benefit is commonly explained in terms of the size of the ice crystals formed during the initial cooling and the effect of warming rate on that size. Higher cooling rates form smaller internal ice crystals, and small crystals appear to be less damaging than large ones. However, if subsequent warming is slow, those small crystals can enlarge to damaging size by the process of recrystallization. Another related possibility is that even at low cooling rates, not all the cell water freezesâa portion may be converted to a glass. If subsequent warming is too slow, this glassy water may devitrify (freeze), with lethal results. This partial glass formation is an introduction to purposeful attempts to induce vitrification of all the cell waterâattempts that are being increasingly pursued in the cryopreservation of tissues and organs, systems that are damaged by extracellular as well as intracellular ice. Vitrification approaches are not covered in this chapter but are treated by Taylor et al. in Chapter 22.
1.2 UNDERLYING BIOPHYSICS AND CELL BIOLOGY
1.2.1 WATER
Water plays a central role in cryobiology and, more important, a central role in biology. Aspects of the latter and their dependence on the unusual and unique properties of liquid water were elegantly described nearly a century ago by L. J. Henderson (1913). Its unusual properties can be seen in a comparison with those of its analog on the periodic table: hydrogen sulfide. For example, the boiling points are 100° and â60°C, respectively; the melting points are 0° and â86°C; the heats of vaporization are 10.7 and 4.5 kcal/mole; and the dielectric constants are 78 and 9 (Lide, 1992; Nemethy and Scheraga, 1964). These properties arise from the structure of the water molecule and its distribution of charge. Infrared and Raman spectroscopy have shown that the water molecule is shaped like an Australian boomerang or a winged seed from a maple tree. The oxygen is at the center of the âboomerang,â and the two hydrogens are located at the tips. The distance between the hydrogen and the oxygen is 0.97 Ă
; the distance between the two hydrogens (i.e., the diameter of the molecule) is 1.54 Ă
(Robinson and Stokes, 1959, p. 2).
The oxygen atom has six electrons in its outer valence shell. Two of these combine with the single electron on the two hydrogen atoms to form the two OâH covalent bonds. These bonds (i.e., the arms of the boomerang) are at an angle of 105 degrees, which is close to the 109 degrees from the center of a tetrahedron to the vertices (Eisenberg and Kauzmann, 1969, p. 4; Robinson and Stokes, 1959, p. 2). The hydrogens bear a net positive charge, with these two positive charges directed toward two of the vertices of a tetrahedron. The remaining four electrons are referred to as âlone-pairâ electrons. The two lone-pair electrons confer a net negative charge to the oxygen side of the water molecule, and these two charges are directed toward the other two vertices of the tetrahedron. Thus, the water molecule bears two net positive charges directed toward two vertices of a tetrahedron and two net negative charges directed toward the other two vertices. An important consequence of this is that the two positive charges on the hydrogen sides of a given water molecule are electrostatically attracted to the lone-pair electrons on the oxygen side of two other water molecules, and the two centers of negativity on the oxygen side of a given water molecule are electrostatically attracted to the protons of two other water molecules. In other words, a given water molecule can be attracted to four other water molecules located at the vertices of the tetrahedron. Two of these surrounding water molecules have their oxygen sides facing the central water molecule, and two of them have their hydrogens facing the central molecule.
The (predominantly) electrostatic attraction between the protons and the oxygens is referred to as the hydrogen bond. Hydrogen bonds are weak (6 to 8 kcal/mole; Eisenberg and Kauzmann, 1969, p. 139) compared, for example, with CâC covalent bonds (145 kcal/mole), but in biological aqueous systems, hydrogen bonds are so numerous as to play a vital role. It is these hydrogen bonds that are responsible for the abnormally high boiling and melting points of water and for its abnormally high heat capacity.
One other important attribute of the water molecule is that the centers of gravity of the two negative charges and the two positive charges do not coincide. This gives water a sizable dipole moment and makes it a polar molecule (Robinson and Stokes, 1959, p. 2). Furthermore, if the water molecule is free to rotate, it possesses a large dielectric constant (78). The large dielectric constant means that it can reduce the electric field between two charged layers or between two charged molecules like ions to a small fraction of what would be the case in a nonpolar solvent.
1.2.1.1 The Structure of Ice
The structure of ice (ordinary Ice I) represents the physical reality of what has just been discussed. That is to say, in ice, each central water molecule is surrounded by, and hydrogen-bonded to, four surrounding water molecules located as though at the vertices of a tetrahedron. The OâO distance is 2.76 Ă
(Eisenberg and Kauzmann, 1969, p. 71). This structure repeats indefinitely. The open structure of ice is responsible for its density, which is much lower than that of most other solids. The extensive hydrogen bonding is responsible for its high heat of fusion and heat of sublimation. The latter is 12.2 kcal/mole and represents mostly the heat necessary to completely break an average of two hydrogen bonds per molecule.
1.2.1.2 The Structure of Liquid Water
The structure of liquid water has been debated for at least 40 years and is still not resolved, but a few generalizations are possible. The structure is similar to ice in that each water molecule tends to be tetrahedrally bound by hydrogen bonds to four other water molecules. To be consistent with the 10% greater density of liquid water at the melting point, however, a liquid water molecule is considered to have ~4.4 nearest neighbors rather than four such neighbors (Robinson and Stokes, 1959, p. 4). It has a short-range structure (i.e., out to about three layers of neighbors) that can be seen by x-ray diffraction, but not the long-range order of ice. The lifetime of a given structure is estimated to be short (i.e., 10â11 sec), although it is relatively long compared with the temporal resolving power of the measuring techniques, which are ~10â13 sec for x-ray and dielectric relaxation.
Models of liquid water structure fall into two broad classes: continuum models and mixture models (Eisenberg and Kauzmann, 1969, p. 254â270; Frank, 1972). Mixture models hypothesize that liquid water consists of a mixture of water molecules forming one, two, three, and four hydrogen bonds and monomeric water forming no hydrogen bonds. Continuum models argue that these discrete classes do not exist, but that liquid water has a distorted hydrogen-bonded structure. The majority of investigators seem to favor the former. The mixture models are further divided into two groups. One model, the interstitial model, hypothesizes that monomeric water molecules reside inside ice-like cages formed by tetrahedrally H-bonded water. These would be analogous to clathrates or gas hydrates in which a nonpolar molecule like methane resides within an ice-like cage. The other mixture model has been picturesquely referred to as the âflickering-clusterâ or âicebergâ theory. It was initially proposed by Frank and Wen (1957) and later elaborated on by Nemethy and Scheraga (1962). It depicts islands or clusters of tetrahedrally H-bonded water lying interspersed in a sea of unbonded water molecules. The lifetime of the clusters is estimated to be 10â10 to 10â11 sec. The argument is that if, as a result of local thermal or energy fluctuations, two water molecules form H-bonds, it becomes energetically favorable for them to form a third and a fourth H-bond. Conversely, if the local fluctuations result in the disruption of an H-bond, it becomes energetically favorable for the other H-bonds to break. Although there appear to be defects in the flickering cluster model (Frank, 1972, p. 533), one attractive feature, a feature that seems to have been ignored by those discussing water models, is that it is consistent with the mechanism proposed for the homogeneous nucleation of supercooled water. In that picture, supercooled water freezes spontaneously when critically sized icelike âembryosâ form in the water as a consequence of energy fluctuations. The number of water molecules that constitute the critical size decreases with temperature, and it is only at about â40°C that the random formation of an âembryoâ with that critical number becomes a probable event (Fletcher, 1962, p. 202).
1.2.1.3 Water around Ions
An attractive electrostatic force exists between the asymmetrical directional charges on a water molecule and ions or ionized groups. The difference between those forces and those in waterâwater interactions is that the orientations of the water molecules are unidirectional around ions. That is, in the first layer of water around a cation, water molecules will tend to be oriented with their negative oxygen sides toward the ion. Around an anion, they will be oriented with their positive protons toward the ion. The attractions can be sufficiently strong to immobilize the water, as manifested in a large decrease in the dielectric constant (Robinson and Stokes, 1959, pp. 15â17).
The forces are short range, affecting, in most ions, only the first layer of water. Small ions such as Fâ or Li+ are an exception in that the effect may extend to the second or third layers. Beyond that, normal water structure is restored. However, as the unidirectional water structure around ions is different from the alternating structure of water, there exists an intermediate region in which water structure is disrupted. Robinson and Stokes (1959, p. 15) point out that when the concentration of an electrolyte solution rises to ~1 M, the second or third water layers from a given ion fall into the sphere of influence of a neighboring ion. As Robinson and Stokes put it, âThe farther from England, the nearer is France.â The net result of these factors is that in most electrolyte solutions, the net water âstructure-breakingâ aspects outweigh those of âstructure making.â
1.2.2 WATER AND MACROMOLECULAR CONFORMATION
The function of macromolecules and complex cellular structures like cell membranes that contain macromolecules depends critically on their conformation. The conformation will be that which minimizes the free energy, and water plays a vital role in that minimization.
1.2.2.1 Maximizing H-Bonds
Hydrogen bonds are not restricted to water. They can form between many groups in macromolecules (Franks, 1973, p. 20) and between these groups and water. This includes the peptide bonds in proteins and the bases in DNA. It is a general tenet that macromolecules will assume a conformation that maximizes the number of internal hydrogen bonds consistent with maximizing the other forces involved. It is important to note, however, that internal hydrogen bonds by themselves do not confer net stability because they are no stronger and, indeed, may be slightly weaker than the hydrogen bonds between the groups and water (Klotz and Franzen, 1962). The most stable conformation will be that wh...
Table of contents
- Cover Page
- Title Page
- Copyright Page
- Foreword
- About the Editors
- Acknowledgments
- Introduction
- Contributors
- Theme 1: Fundamental Aspects
- Theme 2: Life and Death at Low Temperatures
- Theme 3: Freezing and Banking of Living Resources
- Theme 4: Medical Applications
- Theme 5: The Future of Cryobiology