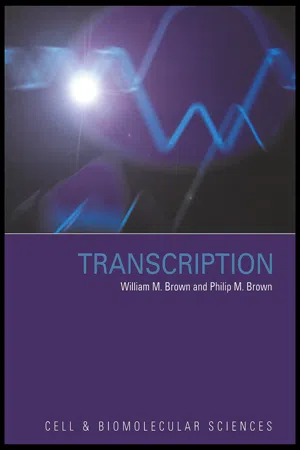
This is a test
- 168 pages
- English
- ePUB (mobile friendly)
- Available on iOS & Android
eBook - ePub
Transcription
Book details
Book preview
Table of contents
Citations
About This Book
Knowledge of transcription has moved forward at a furious pace over recent years, and an understanding of the processes involved in gene regulation and expression has become an essential element in biochemistry, genome biology, molecular biology and molecular genetics. In this timely book, the authors present an accessible, yet comprehensive, cover
Frequently asked questions
At the moment all of our mobile-responsive ePub books are available to download via the app. Most of our PDFs are also available to download and we're working on making the final remaining ones downloadable now. Learn more here.
Both plans give you full access to the library and all of Perlegoâs features. The only differences are the price and subscription period: With the annual plan youâll save around 30% compared to 12 months on the monthly plan.
We are an online textbook subscription service, where you can get access to an entire online library for less than the price of a single book per month. With over 1 million books across 1000+ topics, weâve got you covered! Learn more here.
Look out for the read-aloud symbol on your next book to see if you can listen to it. The read-aloud tool reads text aloud for you, highlighting the text as it is being read. You can pause it, speed it up and slow it down. Learn more here.
Yes, you can access Transcription by William M. Brown, Philip M. Brown in PDF and/or ePUB format, as well as other popular books in Biological Sciences & Cell Biology. We have over one million books available in our catalogue for you to explore.
Information
1
Introduction to Transcription
- Overview
- The central dogma of molecular biology
- Nomenclature
- Selective gene expression
- Other mechanisms involved in transcriptional regulation
OBJECTIVES
- develop an understanding of the scale of the problem of controlling the genome
- introduce the mechanisms and essential components of transcription
1.
OVERVIEW
Consider the genome; it contains genes encoding all the proteins that the cell or organism will ever need. However, at a given point in a cell or an organismâs life cycle, many of these proteins will be unnecessary and it would be wasteful and pointless to express them. In multi-cellular organisms, different cells in different tissues express different subsets of genes. Again, it would be wasteful and pointlessâand even conceivably harmfulâfor a central nervous system neurone to make muscle or liver proteins. How then should the genome be regulated? Should genes be switched âoffâ in the ground state and then switched âonâ when needed, or should genes basically be active and then be switched âoffâ when not needed? Does the answer to that question depend on the size of the genome, the total number of genes, the degree of specialisation of the cells and tissues of the organism, and the fraction of those genes that need to be expressed in a cell at a given time?

Figure 1.1 The âcentral dogmaâ of molecular biology: DNA makes messenger RNA makes protein.
2.
THE CENTRAL DOGMA OF MOLECULAR BIOLOGY
All cells contain a complete genome, that is, the DNA that encodes all the proteins a given organism can ever make. Transcription is the production of RNA from this DNA. Translation is the production of proteins from some of the transcribed RNA, specifically the messenger RNA (mRNA). Such mRNA sequences are also referred to as âtranscriptsâ. Not all proteins are required at all times; how selective gene expression occurs and is regulated is the subject of this book.
2.1
How should transcription be regulated?
The human genome comprises approximately 30,000â40,000 genes; a given cell may require expression of a small proportion of these. Thus, many or even most of the genes may not be required to be expressed at a given time in a given cell.
Many genes in the genome are not expressed at a given time.
How then should the genome be regulated? Should all the genes be constitutively expressed, with expression of many genes being inhibited by some process or mechanism? Alternatively, should expression of all the genes be constitutively prevented, with those that are necessary being specifically activated? What are the implications of these two proposed mechanisms?
In the eukaryotic genome, most genes are âoffâ and are switched âonâ when needed.
When phrased in this manner it seems intuitively obvious that the ground state of a gene should be âoffâ (i.e., not expressed). Then, as and when required, a gene should be switched on for only as long as is necessary, it being extremely wasteful to produce transcripts encoding proteins that are not needed.
While this does seem logical when phrased in this way, it is not, however, a universal answer. Single cell organisms without a nucleus, such as bacteria, are termed prokaryotes. Regulation of gene expression in prokaryotes is largely negative, that is, the genes are âavailableâ for expression but represser molecules switch off genes that are not required for expression. Furthermore, in a single cell organism, with no tissue specialisation, more of the genes of the genome will be required to be expressed.
In prokaryotic genomes, most genes are âonâ and are turned âoffâ when not needed.
It should also be obvious that if all genes were switched âoffâ, the entire genome would be silent, with no gene being transcribed. In this case, there would be no expression of any of the proteins needed to transcribe genes, including RNA polymerase, transcription factors, splicing enzymes, ribosomal proteins or RNAs, the basic enzymes of oxidative metabolism, or anything else. Thus, on reflection it is clear that some gene products will be required by almost all cells at almost all times and perhaps these genes should be constitutively activeâat some levelâso that their gene products are always available in the cell. Additionally, there should also be a mechanism to activate other genes, the products of which will only be needed in some cells some of the time.
However, the basic point that most genes should be inactive most of the time is not lost. It is the most logical and economical manner to regulate the genome, and it is the mechanism evolution has adopted in higher organisms.
3.
NOMENCLATURE
Before proceeding further, this seems a good point to try to clarify the sometimes-confusing nomenclature used in discussing transcription. The following terms have generally accepted meanings that will be adopted throughout the rest of the book.
Sites on DNA are classified by their position in relation to genes and transcription start sites. Thus, the âpromoterâ is the region immediately in front of the transcription start site. This is, therefore, said to be âupstream ofâ or â5â toâ the start site (see Figure 1.2). It is on the promoter that RNA polymerase assembles. Typically, a promoter extends for around 200 base pairs upstream (i.e., bases â1 to â200, there being no base zero). Generally, the eukaryotic promoter contains a TATA box and various other DNA sequences to which proteins (transcription factors) bind, as will be discussed in later chapters.
Enhancers are regions of DNA that may be located upstream or downstream or even in an intron, near the gene or distant from it and in either orientation relative to the transcription start site. Enhancers often contain multiple copies and/or several different types of binding sequences for transcription factors.

Figure 1.2 The first transcribed base is referred to as base +1; this is âdownstreamâ of the promoter. In transcription nomenclature there is no base zero; the last base of the promoter is termed base -1, and the bases âupstreamâ of the transcriptional start site (sometimes referred to as the âtspâ) are numbered negatively.
Promoters and enhancers contain DNA sequences or elements to which DNA-binding proteins bind.
The DNA sequences at which transcription factors bind are variously referred to as elements, sequences, and boxes. The proteins that bind to the sequences are variously referred to as factors, receptors (as in steroid hormone receptors), binding proteins, activators, repressers, or inhibitors.
DNA binding is mediated by distinct domains in transcription factors, some of which can confer DNA-binding activity on proteins that ordinarily do not bind DNA, when joined artificially to them in chimeric proteins.
A transcription factor must have at least two properties, that of DNA binding and activation or repression of gene expression. In addition, transcription factors need to interact with other proteins, especially those of the basal transcription machinery, including RNA polymerase. Other properties seen in transcription factors include ligand binding and nuclear localisation signals.
In many examples, it has been shown that separate domains or regions of the protein mediate the two key properties of DNA binding and gene activation. Such separation of function is most elegantly illustrated by experiments in which domains are swapped or added-using recombinant DNA techniques-to unrelated proteins. The resulting chimeric protein then exhibits the DNA-binding or activating properties of the parent protein.
For example, in the yeast transcription factor âGAL4â the properties can be experimentally separated. GAL4 is a protein that controls the transcription of the genes involved in galactose metabolism in yeast. The protein binds to a yeast enhancer known as the upstream activating sequence (UAS).
GAL4 has three functions; it binds DNA at the UAS sequence, it activates transcription, and it interacts with GAL80, another protein. GAL4 contains three domains, each responsible for one of these functions. These domains function largely independently of each other and can be joined to other proteins and protein domains to give new chimeric proteins with the predicted properties. Thus, when the DNA-binding domain of GAL4 was replaced with that from the protein LexA, the chimeric GAL4 bound only to a reporter gene containing the LexA operator sequence.
Promoter: Specific DNA sequences, usually upstream of a geneâs transcriptional start site, that allow the formation of a transcription complex and allow the initiation of transcription.
Enhancer: Short DNA sequence that can increase the frequency of the initiation of transcription in a largely distance- and orientation-independent fashion.
Intron: A non-coding sequence present within a eukaryotic gene. Introns are spliced out of transcripts during processing to form mature mRNA.
Exon: Part of a eukaryotic gene that is transcribed and usually translated into a protein.
TATA box: A DNA sequence found within prokaryotic promoters, typically
Figure 1.3 Definitions at approximately position â25.
A common technique to simplify the study of transcription is that of using reporter genes attached to the DNA sequence being studied, rather than the endogenous gene to which the promoter is normally connected, A reporter gene is a gene encoding a robust enzyme with a readily quantitated activity, and removes the need for antibodies to the protein of interest or for the protein encoded by the gene to possess enzymatic activity.
For example, the bacterial enzyme -galactosidase is widely used because it converts the colourless substance X-gal (5-bromo-4-chloro-3-indolyl-Ă-Dgalactoside) to a blue product in a simple and versatile, which is easily observed and/or quantitated spectrophotometrically. Similarly, the enzyme chloramphenicol acetyl transferase (CAT) is widely used because its protein product is robust (even in the hands of students!).
The luciferase enzyme from the firefly has also been used. This reporter geneâs protein can generate light from appropriate reagents, and this can be readily measured.
In a more recent development, such reporter genes have been used in combination with transgenic mouse technology to study patterns of expression in the whole animal. The reporter gene, usually -galactosidase, is attached to the promoter of the gene of interest and the transgene is inserted into the mouse genome using a variety of techniques. With luck, in the developing animal, the reporter gene is expressed wherever the endogenous gene would have been expressed, under the control of the same transcription factors.
BOX 1
REPORTER GENES;-GALACTOSIDASE, CAT, LUCIFERASE, AND LAC-Z TRANSGENIC MICE
REPORTER GENES;-GALACTOSIDASE, CAT, LUCIFERASE, AND LAC-Z TRANSGENIC MICE
4.
SELECTIVE GENE EXPRESSION
Even a âsimpleâ single-celled organism, such as the bacterium Escherichia coli (E. coli), contains several thousand genes. The bacterium needs only a subset of these genes to survive in given circumstances while consuming a given carbon source. Additionally the genes needed to be expressed will change throughout the organismâs life cycle and will depend on the bacteriumâs environment.
In multi-cellular organisms the control of gene expression must become more complex, simply because the genome is larger and the life cycle more complex. In the multi-cellular organism, each cell contains the same genome (the same genotype), but different cells within the organism will be specialised, will have different func...
Table of contents
- Cover Page
- Cell and Biomolecular Sciences
- Title Page
- Copyright Page
- Series Preface
- Abbreviations
- 1: Introduction to Transcription
- 2: Transcription, the Process
- 3: Transcription Factors in Human Diseases
- 4: The Components of Transcription
- 5: Bacterial Transcription
- 6: Eukaryotic Transcription
- 7: Chromatin and Eukaryotic DNA
- 8: Bacteriophage Lambda ( ): A Transcriptional Switch
- 9: The Lactose Operon in Escherichia Coli
- 10: Other Transcription Systems, Eukaryotic and Prokaryotic
- Glossary
- End of Unit Questions and Answers