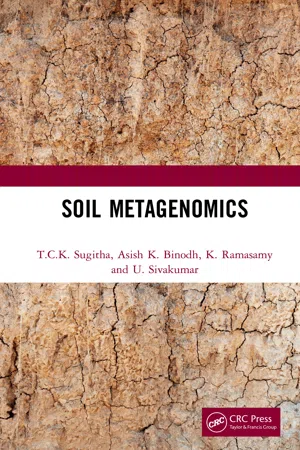
This is a test
- 258 pages
- English
- ePUB (mobile friendly)
- Available on iOS & Android
eBook - ePub
Soil Metagenomics
Book details
Book preview
Table of contents
Citations
About This Book
This book focuses on the recent advents and technological breakthroughs in metagenomic approaches coupled with their applications in agriculture. The intended audience include soil and environmental microbiologists, molecular biologists and policy makers. The book expertly describes the latest fourth generation metagenomic technologies from sample collection to data analysis, metatranscriptomic, metaproteomic and metabolomics studies
Note: T& F does not sell or distribute the Hardback in India, Pakistan, Nepal, Bhutan, Bangladesh and Sri Lanka.
Frequently asked questions
At the moment all of our mobile-responsive ePub books are available to download via the app. Most of our PDFs are also available to download and we're working on making the final remaining ones downloadable now. Learn more here.
Both plans give you full access to the library and all of Perlego’s features. The only differences are the price and subscription period: With the annual plan you’ll save around 30% compared to 12 months on the monthly plan.
We are an online textbook subscription service, where you can get access to an entire online library for less than the price of a single book per month. With over 1 million books across 1000+ topics, we’ve got you covered! Learn more here.
Look out for the read-aloud symbol on your next book to see if you can listen to it. The read-aloud tool reads text aloud for you, highlighting the text as it is being read. You can pause it, speed it up and slow it down. Learn more here.
Yes, you can access Soil Metagenomics by T.C.K. Sugitha, Asish K. Binodh, K. Ramasamy, U. Sivakumar in PDF and/or ePUB format, as well as other popular books in Technologie et ingénierie & Agronomie. We have over one million books available in our catalogue for you to explore.
Information
CHAPTER 1
INTRODUCTION TO METAGENOMICS
Soil is a living organ of the earth. Until recent times large and small civilizations throughout the world recognized this truth, expressing it through a deep spiritual relationship with soil. The Old Testament in Bible describes the soil as a source of healing. “The Lord hath created medicines out of the earth, and he that is wise will not abhor them” (Ecclesiasticus 38:4). Even in New Testament of Bible narrates an incident where Jesus Christ healed a blind man by putting a paste made from soil on the man’s eyes (John 9). The ancient Greeks worshipped soil through the goddesses Gaia and Demeter, the Germans through goddesses Ertha and the Native Americans through Mother Earth.
Advances in science, medicine and agriculture during the 20th century provided numerous reasons to continue to venerate the soil well beyond the basic necessity of food production. Discovery during this century about soil biology led to the development of life saving drugs including antibiotics, antitumour agents and immune-suppressants. And yet, just as these advances grew more impressive, urbanization and other global economic influences tended to disassociate many people from an intimate association with the environment and gradually we lost appreciation of our reliance on the soil. But that trend may be altered by the renaissance in soil biology that presents the soil as one of the last great frontiers available for discovery and bioprospecting. This renaissance is driven by the tools of molecular biology, which enable exploration of the life and chemistry of soil in new ways. One emerging tool that draws together traditional soil biology and modern molecular biology is metagenomics which entails collective analysis of genomes of an assemblage of microorganisms.
1.1. SOIL AS A MICROBIAL HABITAT
Soil comprises mineral particles of different sizes, shapes and chemical characteristics, together with the soil BIOTA and organic compounds in various stages of decomposition. The biological processes in soil are executed in a complex physical and chemical environment. The physical structure of soil is varied and dynamic soil particles vary in size from 1 to 1000 micrometers and the moisture content and chemistry of soil affect particle size. The particle size inturn affects water flow, gas exchange and temperature gradient in soil. The chemical composition of soil is derived from a combination of its geologic and biologic origins.
The formation of clay–organic matter complexes and the stabilization of clay, sand and silt particles through the formation of aggregates are the dominant structural characteristics of the soil matrix. Soil-matrix-component aggregates range from approximately 2 mm or more (macro aggregates) to fractions of a micrometer for bacteria and colloidal particles.
Prokaryotes are the most abundant organisms in soil and can form the largest component of the soil biomass. Soil microorganisms often strongly adhere or adsorb onto soil particles such as sand grains or clay–organic matter complexes. Microhabitats for soil micro organisms include the surfaces of the soil aggregates, and the complex pore spaces between and inside the aggregates. Some pore spaces are inaccessible for microorganisms owing to size restrictions.
1.1.1. Soil moisture and Microbial biomass
The metabolism and the survival of soil microorganisms are strongly influenced by the availability of water and nutrients. In contrast to aquatic habitats, surfaces of soil environments undergo dramatic cyclic changes in water content, ranging from water saturation to extreme aridity. A fraction of the microbial community dies during each drying-and-wetting cycle. As a consequence, the composition of soil microbial communities fluctuates.
1.1.2. Microbial biomass and biogeochemical cycles
Microorganisms active in the soil are largely responsible for biogeochemical cycles that support life on earth. Soil is an important reservoir for organic carbon, and prokaryotes are an essential component of the soil decomposition system. Despite the high concentration of organic matter in most soil types, only low concentrations of organic carbon are readily available to microorganisms. Reasons for this include the transformation of most of the organic matter that is derived from plants, animals and microorganisms into humus by a combination of microbiological and abiotic processes, and the uneven distribution of microorganisms and organic compounds in the soil matrix. Humic substances are stable and recalcitrant to microbial decomposition processes the half-life of these stable organic matter complexes with respect to biological degradation is approximately 2,000 years. Since many of these changes are driven by microorganisms the structure and function of the microbial communities get affected.
Apart from Carbon cycle in soil, microorganisms also mediate the Nitrogen cycle, Sulphur cycle, oxidative and reductive transformation of metals such as iron, silica and mercury. Soil microorganisms also influence the health of plants and animals by providing vitamins and other nutrients, thereby influencing the developmental process. Microorganisms exist within a carefully balanced natural ecosystem of checks and balances and when disrupted through human agency can produce harmful environmental effects. Hence the microbial diversity and the corresponding gene pool, must be large.
1.2. HISTORY OF SOIL METAGENOMICS
Ever since Robert Koch established his pioneering postulates on the microbial nature of disease, the field of microbiology has centered on the process of cultivating individual microbial species. Growing an organism in pure culture has been the critical first step towards understanding the properties of a given microbe. This culture dependent, reductionist approach has produced many impressive successes of microbiology in the 20th century. However, the limitations of culture dependent studies were first recognized by what has become known as the ‘great plate count anomaly’. The advent of new molecular and microscopic methods has fostered a growing realization, however, that there is much to be known about soil microorganisms that cannot be elucidated through traditional approaches. At the heart of this revolution was the convincing demonstration that the uncultured microbial world far outsized the cultured world and that this unseen world could be studied.
In 1931, Waksman optimistically believed that “a large body of information has accumulated that enables us to construct a clear picture of the microscopic population of the soil”, and in 1923 Bergey’s Manual stated categorically that no organism could be classified without being cultured. Pace and colleagues highlighted the need for nontraditional techniques to understand the microbial world: “The simple morphology of most microbes provides few clues for their identification; physiological traits are often ambiguous. The microbial ecologist is particularly impeded by these constraints, since so many organisms resist cultivation, which is an essential prelude to characterization in the laboratory”. In the ensuing years, microbiologists dedicated intense effort to describe the phylogenetic diversity of exotic and ordinary environments ocean surfaces, deep sea vents, hot springs, soil, animal rumen and gut, human oral cavity and intestine. Many new lineages were classified based on their molecular signatures alone. The next challenge was to elucidate the functions of these new phylotypes and determine whether they represented new species, genera, or phyla of prokaryotic life. This challenge spawned various techniques, including metagenomics, the genomic analysis of assemblages of organisms. In a few years, the study of uncultured microorganisms has expanded beyond asking “Who is there?” to include the difficult question “What are they doing?.” The recent advent of pyrosequencing and next generation sequencing technologies unlocked the magics and menaces of microbes present in a given environment. Some of the challenges that opened the concept of ‘uncultured world’ are discussed below.
1.2.1. Sulfolobus from Yellowstone hot springs
From the 1880s forward, the microbiological world was divided into the cultured and the uncultured. It is very obvious that culturing did not capture the full spectrum of microbial diversity. One of the indicators that cultured microorganisms did not represent much of the microbial world was the oft-observed “great plate count anomaly” the discrepancy between the sizes of populations estimated by dilution plating and by microscopy. Brock and colleagues encountered microorganisms in Yellowstone hot springs that could not be cultured and others whose behavior in culture did not reflect their activities insitu. Due to the Yellowstone Plateau’s high elevation the average boiling temperature at Yellowstone’s geyser basins is 199 °F (93 °C). Water erupting from Yellowstone’s geysers is superheated above that boiling point to an average of 204 °F (95.5 °C) as it leaves out the vent. Many of the organisms could not be cultured on agar medium because their temperature requirements exceed the melting point of the agar. Brock’s central technique involved the immersion of microscope slides in the spring for 1 to 7 days, followed by microscopic examination and often staining with fluorescent antibodies raised against cultured members of the taxonomic groups suspected to inhabit the environment. This approach estimated in situ population sizes and growth rates, which indicated, for example, that certain strains of Sulfolobus grew in the hot springs at temperatures well below the optima in pure culture. Further Brock’s group inferred that the genus Synechococcus, typically pink in culture, was a major contributor to photosynthesis in hot springs.
1.2.2. Vibrio cholera – the challenging food pathogen
Further evidence that drew attention to the uncultured world accumulated during the 1970s and 1980s. A study of oligotrophs indicated that incubation times longer than 25 days enhanced the recovery of certain organisms in culture. The food industries generated intense interest in “injured bacteria” in food live organisms that cannot be cultured following stressful treatments such as heat, chilling, or desiccation but represent a significant risk to human health The concept of organisms that were viable but not culturable emerged from the work of Colwell and colleagues, who showed that strains of Vibrio cholerae were indeed alive and virulent when isolated from aquatic environments but did not grow in culture until after passage through a mouse or human intestine.
1.2.3. The dreadly Helicobacter Pyroli
The confluence of these and many other scientific and technical advances steadily drew attention to the unculturable microbial world, but two discoveries figured significantly in the sharpened focus. i) The diversity of soil bacteria, which demonstrated with DNA-DNA reassociation techniques confirmed that the complexity of the bacterial DNA in the soil was at least 100-fold greater than could be accounted for by culturing. ii) The second discovery was the demonstration that Helicobacter pylori causes gastric ulcers and cancer. Although spiral bacteria had been observed in the gastric mucosa of dogs in 1893 and in humans in 1906, the correlations between the appearance of the bacteria and peptic ulcers were noted in 1938 untill H. pylori was cultured. These discoveries provided compelling evidence that drew microbiologists to wrestle with the daunting challenge of devising strategies to access these organisms.
1.2.4. A shift in the Paradigm
In 1985, an experimental advance radically changed the way we visualize the microbial world. Building upon the pioneering work of Carl Woese, which showed that rRNA genes provide evolutionary chronometers, Pace and colleagues created a new branch of microbial ecology. They used direct analysis of 5S and 16S rRNA gene sequences in the environment to describe the diversity of microorganisms in an environmental sample without culturing.
The next technical breakthrough arrived with the development of PCR technology and the design of primers that can be used to amplify almost the entire gene. This accelerated the discovery of diverse taxa as habitats across the earth. The application of PCR technology provided a view of microbial diversity that was not distorted by the culturing bias and revealed that the uncultured majority is highly diverse and contains members that diverge deeply from the readily culturable minority. Today, 52 phyla have been delineated, and most are dominated by uncultured organisms (Fig. 1.1).
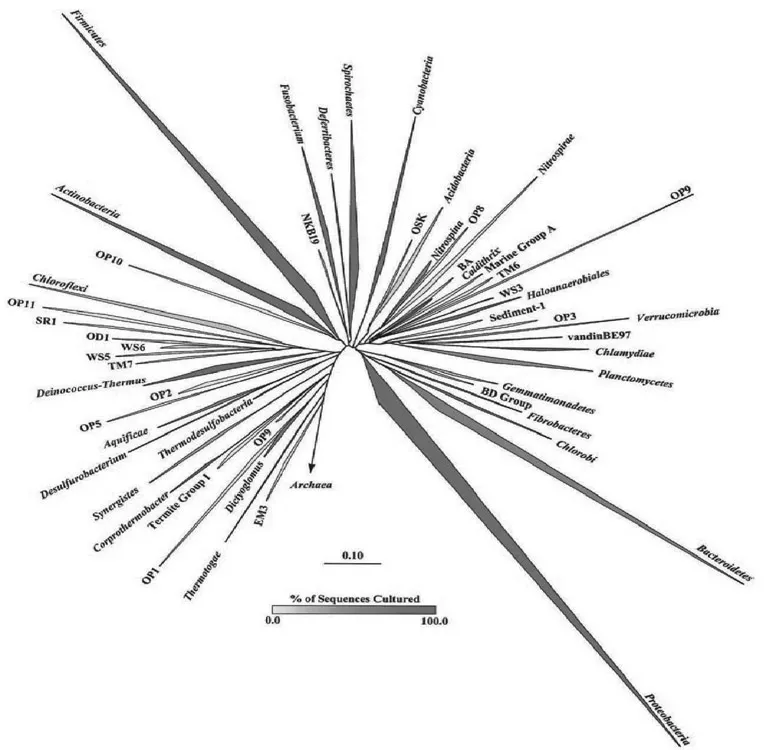
Fig. 1.1. Phylogenetic tree of Bacteria showing established phyla (italicized Latin names) and candidate phyla. The vertex angle of each wedge indicates the relative abundance of sequences in each phylum; the length of each side of the wedge indicates the range of branching depth found in that phylum; the redness of each wedge corresponds to the proportion of sequences in that phylum obtained from cultured representatives. Candidate phyla do not contain any cultured members.
The application of phylogenetic stains (Fig. 1.2) nucleic acid probes with fluorescent labels facilitate visualization of single cells insitu where as traditional microscopy provides little phylogenetic information. Fluorescent antibody studies require prior knowledge and culturing of an organism or one closely related to it to raise antibodies. Phylogenetic stains require only an rRNA sequence, which can be derived from an environmental sample without culturing.
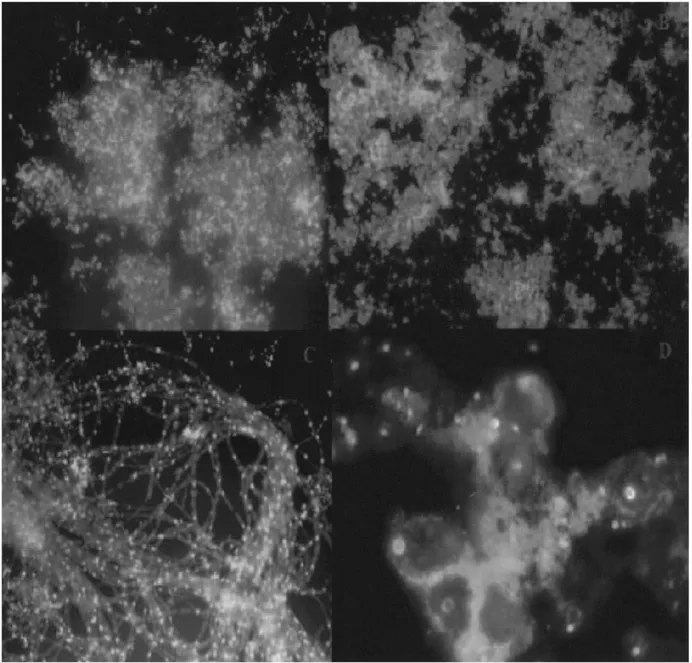
Fig. 1.2. Phylogenetic stains. Fluorescent in situ hybridization biofilm samples from Iron Mountain Mine, Calif. Nucleic acid probes were labeled with indodicarbocyanine, and DNA was stained nonspecifically with 4_,6_-diamidino-2-phenylindole. The nucleic acid probes are specific for (top left) Sulfobacillus spp., (top right) Archaea, (bottom left) Archaea on fungal filaments, and (bottom right) Eukarya.(Courtesy:Baker et al., 2003)
Pace and his colleagues during the year 1991, first proposed the idea of cloning DNA directly from environmental samples and in 1991, the first such cloning in a phage vector was reported. The next advance was the construction of a metagenomic library with DNA derived from a mixture of organisms enriched on dried grasses. Clones expressing cellulolytic activity were found in these libraries, which were referred to as zoolibraries. The work of DeLong’s group reported libraries constructed from prokaryotes in seawater and identified a 40-kb clone that contained a16S rRNA gene indicating that the clone was derived from an archaeon that had never been cultured. Construction of libraries with DNA extracted from soil lagged due to difficulties associated with maintaining the integrity of DNA during its extraction and purification from a soil matrix but eventually produced analyses analogous to those from seawater.
1.2.5. rRNA Analysis and Culturing
In addition to providing a universal culture-independent means to assess diversity, 16S rRNA sequences also provided an aid to culturing efforts. Bacteria remains recalcitrant to culturing for diverse reasons—lack of necessary symbionts, nutrients, or surfaces, excess inhibitory compounds, incorrect combinations of ...
Table of contents
- Cover
- Half Title
- Title Page
- Copyright Page
- Contents
- Preface
- 1. Introduction to Metagenomics
- 2. Soil Metagenomics - The Culture Independent Insight
- 3. Techniques in Soil Metagenomics
- 4. Accessing the Soil Metagenome
- 5. Construction of Soil Metagenomic Libraries
- 6. Enrichment Strategies of Soil Metagenome
- 7. Metagenomic Pyrosequencing and Microbial Identification
- 8. Metagenomic Gene Discovery
- 9. Taxonomic and Functional Diversity of Microbial Communities
- 10. Metagenomics and Ecology
- 11. Mobile Metagenomics
- 12. Viral Metagenomics – Generation of Viral Metagenome
- 13. Mapping the Virus World Through Metagenomics
- 14. Functional Viral Metagenomics
- 15. Metagenomics and Integrative ‘Omics’ in Bioremediation
- 16. Metagenomics in White Biotechnology
- 17. Metagenomics in Agriculture
- 18. Bioinformatics in Metagenomics
- 19. Nanotechnology in Metagenomics
- 20. The Future of Metagenomics
- Glossary
- Bibliography